Key Concepts in Physics
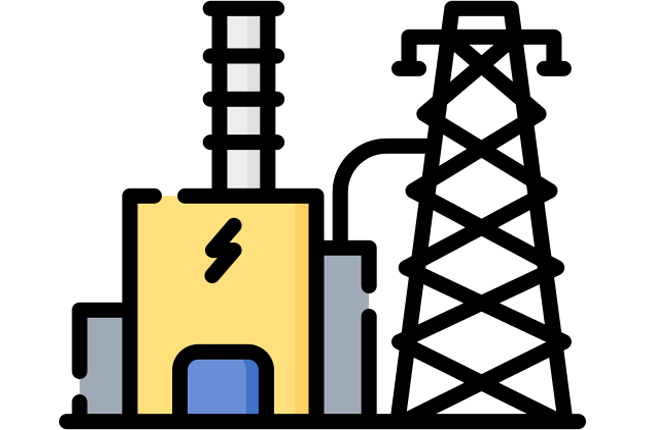
Energy & Power
Physics Essentials – Concepts and Practical Examples
Physics stands at the core of countless discoveries and technological breakthroughs, shaping how people interpret the behavior of matter, energy, and the fundamental interactions that govern the universe. In many ways, it serves as the foundation for all other sciences, offering explanations that clarify everything from everyday motion to the mysteries of distant galaxies. Below is a thorough exploration of essential physics principles, highlighting core ideas and their significance for modern technology, engineering, and daily life.
Newtonian Mechanics
Newtonian mechanics, developed by Sir Isaac Newton, forms the foundation of classical physics. It describes the motion of objects and the forces acting upon them.
Laws of Motion
Newton’s three laws of motion explain how objects move and interact. The first law, also known as the law of inertia, states that an object at rest stays at rest, and an object in motion stays in motion unless acted upon by an external force.
The second law relates force, mass, and acceleration, providing the equation
The third law states that for every action, there is an equal and opposite reaction.
In everyday life, these laws explain everything from how cars accelerate and brake to why we feel a pushback when we push against a wall.
Kinematics and Dynamics
Kinematics concentrates on describing motion through parameters such as velocity, displacement, and acceleration, without identifying the causes behind that motion. This outlook provides insights into constant acceleration scenarios, including objects dropped under gravity or projectiles thrown into the air. Meanwhile, dynamics aligns closely with Newton’s second law by analyzing how forces cause motion to alter. Engineers depend on these perspectives when conceiving safe transportation systems, ensuring vehicles can accelerate and brake under predictable force distributions, or refining the flight paths of aircraft, missiles, and satellites.
Circular Motion and Centripetal Force
Many natural and engineered systems involve circular paths, such as planets orbiting the Sun or electrons traveling around an atom’s nucleus. The concept of centripetal force emerges whenever an object’s direction changes while maintaining constant speed. This inward-pulling force keeps the path curved, rather than letting an object fling outward. Designers of roller coasters calculate centripetal forces to keep riders pressed safely against their seats during sharp loops, while scientists interpret how satellites remain in stable orbits around Earth. The same principle guides the use of centrifuges in laboratories to separate substances based on density.
Work, Energy, and Power
Work signifies the product of force applied over a distance, representing how energy is transferred to or from a system. When individuals climb stairs or compress a spring, they are doing work against gravitational or elastic forces. Energy appears in numerous forms—kinetic, potential, thermal—and can transform from one type to another without any net loss or gain in the total. Power measures how rapidly work is completed, an aspect relevant in mechanical systems and electrical circuits. Recognizing these relationships helps engineers design everything from electric motors to household appliances with efficiency in mind.
Conservation of Momentum
In a closed system, total momentum remains constant unless external forces enter the picture. Momentum unites mass and velocity, providing a tool to study interactions like collisions, explosions, or recoil effects. Sports enthusiasts rely on momentum strategies in soccer or basketball, gauging how best to direct the ball for optimal distance or to maneuver around defenders. Vehicle safety also hinges on momentum analyses that inform the creation of crumple zones, allowing collisions to dissipate kinetic energy more safely.
Thermodynamics
Thermodynamics examines how heat, work, temperature, and energy interconnect. This field underlies power generation, refrigeration, and the study of why processes spontaneously flow in certain directions.
Laws of Thermodynamics
Three principal laws anchor thermodynamic behavior. The first law says energy cannot be created or destroyed, only transformed from one form to another—a restatement of the principle of energy conservation. The second law recognizes that processes naturally move toward increased disorder, captured by the term entropy, meaning energy disperses if no external force redirects it. The third law posits that as a system cools toward absolute zero in temperature, its entropy approaches a definitive minimum. Power plants, automobiles, and air conditioners rely on these rules, transforming heat into mechanical work or maintaining comfortable ambient conditions.
Heat Transfer
Heat travels between bodies via conduction, convection, or radiation. Conduction involves direct contact, evident when a metal spoon left in a hot pot becomes warm. Convection appears in fluid flow patterns, such as warm air currents circulating inside a room heated by a radiator. Radiation involves electromagnetic waves, exemplified by the warmth felt from the Sun. Each mechanism shows up in daily settings, from cooking methods in a kitchen to the design of specialized devices like heat exchangers and cooling towers.
Thermal Expansion
Most materials expand when heated, a phenomenon that influences engineering solutions. Bridges, for example, include expansion joints to accommodate changes in length, preserving structural stability. Glass cookware must endure sudden shifts in temperature, or else thermal stress can cause cracks. Knowledge of coefficients of expansion helps predict and control these structural challenges, ensuring safe performance in wide-ranging weather or processing conditions.
Entropy and Disorder
Entropy represents the measure of randomness or disorder, clarifying why spontaneous events lean toward more spread-out energy states. Ice cubes melt in a warm room, left unchecked, because the system plus its surroundings experiences a net rise in entropy. Industrial planners consider these trends when seeking optimal operating temperatures for chemical reactions, ensuring that processes move forward efficiently without incurring wasted energy or resources.
Electricity and Magnetism
Electromagnetic phenomena support virtually every modern convenience, from lighting and household appliances to telecommunications and advanced computing hardware.
Electric Charge and Electric Field
Objects gain electric charge when electrons are lost or gained, causing them to exert forces on one another. Static electricity on clothing or doorknobs highlights charge accumulation, although practical applications stretch much further. Photocopy machines exploit electrostatic forces to position toner on paper, and electrostatic precipitators remove pollution from smokestack gases. Electric fields define how charges act at a distance, giving rise to concepts like voltage.
Electric Potential and Voltage
Electric potential measures how much work each unit of charge can perform if freed to move, while voltage gauges potential differences between two points in a circuit or region of space. It shows up in everyday batteries, where chemical processes set up a voltage difference across terminals, driving electron flow through connected devices. Electric vehicles rely on stacks of lithium-ion cells that deliver the necessary voltage and current. On a larger scale, power plants dispatch electrical energy along distribution grids at high voltage to reduce losses from resistance over long distances.
Current, Resistance, and Ohm’s Law
Current defines the rate at which charge flows, measured in amperes, while resistance quantifies how much a material opposes the movement of those charges. Ohm’s law, stated simply as , shows how voltage, current, and resistance interrelate. Electronics design depends on these principles to ensure that components like resistors, diodes, and transistors behave predictably and do not burn out under operating conditions. Household wiring codes revolve around safe current levels to avoid overheating or short-circuits.
Series and Parallel Circuits
In series circuits, components are connected end-to-end, so the same current flows through each. In parallel circuits, components are connected across common points, allowing multiple paths for the current.
Knowing how to design and analyze series and parallel circuits is essential for electricians and engineers working with complex electrical systems.
Magnetic Fields and Electromagnetism
Magnetic fields arise from moving charges or inherent magnetic moments in materials. Conductors carrying current produce surrounding fields, influencing compasses or other ferromagnetic objects. Electromagnets rely on coils of wire around iron cores to generate powerful magnetic effects, vital in everything from scrap-metal salvage to high-speed maglev trains. The synergy between electricity and magnetism emerges in the concept of electromagnetic induction, where changing magnetic flux through a coil induces an electric current. Generators, found in wind turbines or hydroelectric dams, harness induction by converting mechanical energy into electrical energy.
Faraday’s Law of Induction
Faraday’s law states that a changing magnetic field induces an electric current in a conductor. This principle is the basis for electric generators and transformers.
Wind turbines and hydroelectric dams use Faraday’s law to convert kinetic energy into electrical energy, providing renewable power sources.
Alternating Current (AC) and Direct Current (DC)
Charges in DC systems move steadily in one direction, whereas AC toggles polarity many times per second. AC remains the standard for household outlets, as it travels efficiently over long power lines and is compatible with transformers that modify voltage levels. DC thrives in portable electronics and automotive systems. Engineers unify AC and DC in advanced power grids, ensuring stable electricity delivery to industries and consumers while bridging diverse energy sources like solar panels or wind farms.
Waves and Optics
Wave behavior governs how energy and signals travel through materials or across empty space. From audible sounds to visible light, wave theory provides methods to analyze frequency, amplitude, and propagation.
Properties of Waves
Wavelength measures the distance between successive wave crests, frequency counts how many crests pass a point per second, and amplitude defines the wave’s height or intensity. Together, these attributes shape how waves transfer energy. Radio broadcasts, for instance, differ from visible light or microwaves only in frequency and wavelength. Communication networks rely on carefully assigned wave bands to avoid interference, enabling stable phone calls, satellite links, or data streaming.
Sound Waves and Acoustics
Sound arises from pressure variations traveling through mediums such as air or water. Acoustics examines these vibrations, including how they reflect or diffuse in enclosed rooms. Theater architects design concert halls guided by acoustic research, seeking to minimize echoes while keeping musical notes crisp. Ultrasound technology harnesses high-frequency sound waves to visualize internal body structures, operating on the principle that different tissues reflect sound differently.
Light Waves and the Electromagnetic Spectrum
Electromagnetic waves incorporate radio waves, microwaves, infrared, visible light, ultraviolet, X-rays, and gamma rays. Each group interacts uniquely with matter. Microwaves heat food by agitating water molecules, while ultraviolet radiation triggers tanning or sunburn. X-rays penetrate soft tissues but not denser materials like bone, making them invaluable for diagnostic imaging. Understanding wave interactions propels innovations in remote sensing, astronomy, or medical treatments such as targeted radiation therapy.
Reflection, Refraction, and Diffraction
Light can bounce off reflective surfaces, bend when traveling between media of differing optical densities, or spread out when passing around obstacles or through narrow openings. These wave phenomena inform lens design for cameras, telescopes, and eyeglasses, ensuring correct focus and minimal distortion. Optics also drives research on fiber-optic cables used in internet data transmission, where light signals carry vast amounts of information across continents.
Lenses and Mirrors
Lenses and mirrors manipulate light to form images. Convex and concave lenses focus and diverge light, respectively, while mirrors reflect light.
Optical devices like microscopes, telescopes, and cameras rely on the principles of lenses and mirrors to magnify and capture images of distant or tiny objects.
Optical Instruments
Devices such as microscopes, telescopes, and spectrometers rely on optical principles to expand human observational capacity. Microscopes magnify tiny structures in biology and material science, unveiling cell components or micro-crystals. Telescopes gather dim light from celestial objects, revealing distant galaxies and star-forming regions. Spectrometers break light into component wavelengths, indicating an object’s chemical composition, temperature, and velocity. Collectively, these instruments have unlocked knowledge about microscopic molecular arrangements, as well as the grand scale of cosmic events.
Modern Physics
While classical physics explains many everyday phenomena, breakthroughs in the early twentieth century ushered in relativity and quantum mechanics, reshaping our understanding of space, time, and the subatomic realm.
Relativity
Albert Einstein’s theories of special and general relativity altered concepts of time and gravity. Special relativity clarifies how objects moving near the speed of light experience time dilation and length contraction, validated experimentally by high-energy particle accelerators. General relativity interprets gravity not as a force but as a curvature of spacetime, predicting phenomena like gravitational lensing, where massive objects bend light passing nearby. Practical uses appear in Global Positioning System (GPS) satellites, which adjust for relativistic effects to maintain precise navigation data.
Quantum Mechanics
Quantum mechanics addresses matter and energy on atomic and subatomic scales. Rather than acting like tiny billiard balls, particles display wave-particle duality and often exist in superpositions of states until measured. These concepts underpin lasers, transistors, and diodes, enabling everything from digital displays to high-speed data processing in modern electronics. Quantum entanglement and superposition continue to intrigue researchers working on quantum computing, an endeavor that may transform encryption, artificial intelligence, and large-scale simulations.
Atomic Models and Spectroscopy
Scientists who investigate atomic structures rely on spectroscopic methods to see how electrons absorb or emit light at specific frequencies. This approach indicates energy levels within an atom and reveals which elements are present in distant stars. Astronomers, by capturing spectral lines in the light from galaxies, can infer their motion relative to Earth, providing key support for theories about cosmic expansion. On a smaller scale, labs employ spectroscopy to identify unknown compounds or measure pollutant concentrations in air and water.
Nuclear and Particle Physics
Atomic nuclei hold protons and neutrons bound by the strong nuclear force. This domain brings forth radioactivity, nuclear fission, and nuclear fusion, phenomena harnessed in medical diagnostics, power generation, and potential future energy systems. Particle accelerators like the Large Hadron Collider probe fundamental constituents, investigating quarks, leptons, bosons, and the interactions that shape them. Breakthroughs in this area shed light on how the universe emerged and whether undiscovered particles still await detection.
Applications of Quantum Mechanics
Quantum mechanics is not just a theoretical discipline; it has profound real-world applications. Lasers, which are ubiquitous in modern technology, from barcode scanners to eye surgery, operate on principles derived from quantum mechanics. Semiconductors, the building blocks of all modern electronics, are also based on quantum principles, which allow us to manipulate the flow of electrons in devices like computers and smartphones.
Fluid Mechanics
Liquids and gases exhibit unique behaviors that influence aerodynamics, hydrostatics, and the performance of pipelines, pumps, or turbines.
Properties of Fluids
Fluids do not possess a fixed shape, conforming instead to containers or flow patterns. Density, viscosity, and pressure vary across fluid types and conditions, shaping how liquids or gases respond to forces. The design of water supply networks, for example, depends on analyzing flow rates, pipe diameters, and pump capacities to guarantee consistent delivery. Meteorology also relies on fluid dynamics to forecast atmospheric conditions.
Buoyancy and Archimedes’ Principle
The phenomenon of buoyancy emerges because fluid pressure is greater at deeper levels, producing a net upward force on submerged objects. Archimedes’ principle states that the buoyant force equals the weight of fluid displaced. This concept enables shipbuilders to determine whether a vessel will float properly. Submarines manipulate their buoyancy by adjusting ballast tank contents, controlling depth. Meanwhile, hot-air balloon pilots raise altitude by heating air inside the balloon, decreasing density relative to cooler air outside.
Fluid Dynamics
Flows can be laminar—smooth and orderly—or turbulent—chaotic and swirling. Energy losses from friction, known as head loss, matter in designing pumps or controlling drag on airplanes. Bernoulli’s principle ties fluid velocity to pressure, explaining how airplane wings generate lift by causing air to accelerate over the wing’s top surface. Airfoils in wind turbines follow similar logic, capturing energy from moving air to spin blades that drive generators. Additional branches of fluid dynamics pertain to weather modeling or analyzing blood flow in arteries.
Circular and Rotational Motion
Spinning systems add another layer of complexity beyond linear motion. Many industrial machines revolve, from simple wheels to sophisticated turbines.
Rotational Kinematics
When objects rotate, angles replace the role of linear displacement, with angular velocity and angular acceleration describing how quickly a body spins. Knowing these measures allows engineers to set safe operating speeds, as in a car engine’s crankshaft or a factory’s conveyor belts. Calculating rotational trajectories also influences robotic arms or rotating platforms in space stations that create artificial gravity.
Torque and Angular Momentum
Torque appears whenever a force causes an object to rotate around a pivot, with the force’s magnitude and lever arm distance playing essential roles. Angular momentum parallels linear momentum but applies to rotating bodies, such that rotating objects tend to continue spinning at a constant rate unless external torques act. Professional divers utilize angular momentum conservation to execute flips and twists midair, while rotating machinery parts must balance loads carefully to avoid vibrational damage. Understanding torque is also crucial for automotive engineers who want to optimize engines for power and reliability.
Rotational Inertia
A distribution of mass relative to the axis of rotation shapes how strongly an object resists changes in spin, known as rotational inertia or moment of inertia. This factor explains why rotating ice skaters can alter spin speed dramatically by pulling in their arms, minimizing rotational inertia and boosting angular velocity. Designers of flywheels in mechanical systems or spinning discs in data storage exploit rotational inertia for energy smoothing or data reliability.
Gravitation
Gravity emerges as a universal attraction that influences orbital paths, planetary systems, and everyday experiences like dropping objects to the ground.
Newton’s Law of Universal Gravitation
Newton’s universal law states that every mass exerts an attractive force on other masses, governed by the product of their masses and inversely by the square of the distance between them. Earth orbits the Sun rather than wandering off into space because of this mutual pull, while tides fluctuate because the Moon’s gravitational tug shifts water across Earth’s surface. In satellite technology, engineers rely on gravitational equations to set launch velocities and choose stable orbits for communications or observations.
Gravitational Fields and Potential Energy
Large masses generate a gravitational field around them, dictating how other objects accelerate when nearby. Gravitational potential energy arises when an object’s position allows it to fall or move under gravity, as with roller coasters cranking upward then descending at thrilling speeds. Architectural stability depends on factoring gravitational loads, ensuring that tall buildings remain structurally sound against wind and seismic forces. Astronomical bodies, from dwarf planets to gargantuan black holes, exhibit gravitational fields that shape cosmic architecture.
Orbital Motion
Planets, moons, and artificial satellites trace elliptical orbits, balancing forward inertia with gravitational pull. Space agencies plan missions around these pathways, timing rocket burns so spacecraft achieve stable trajectories or intercept other celestial bodies. The geometry of Earth’s orbit around the Sun, slightly elliptical, impacts seasonal changes. Physics labs replicate orbital concepts on smaller scales, studying ring-like phenomena or cyclotrons used for particle acceleration.
Materials and Properties
Each substance demonstrates characteristic mechanical and electrical traits that dictate its best uses.
Elasticity and Hooke’s Law
Elastic materials revert to original forms when stretched or compressed within certain limits, described mathematically by Hooke’s law. Springs in car suspension or mattresses obey this principle as they store and release mechanical energy. Construction materials must remain elastic enough to handle shifting loads, yet not so flexible that they cannot support required weights.
Stress, Strain, and Material Behavior
When forces act on a solid, stress describes the distribution of force across its area, while strain measures the resulting deformation. Engineers examine these parameters to confirm whether a structure can handle anticipated loads, choosing between materials like steel, aluminum, or polymers. In advanced applications, composites with tailored stress resistance create lighter, stronger aerospace components.
Properties of Materials
Hardness, conductivity, melting point, ductility, and brittleness define whether an element or alloy can endure heat, conduct current, or deform under tension. Silicon’s semiconducting nature drives microchips, while copper’s excellent conductivity shapes electrical wiring. High-temperature alloys keep jet turbines functional, and specialized ceramics withstand harsh chemical environments in industrial reactors.
Energy and Power
Progress in technology hinges on transforming one form of energy to another, from mechanical motion to electrical flow or from sunlight to stored chemical potential.
Kinetic and Potential Energy
An object in motion carries kinetic energy that depends on its mass and speed, while potential energy arises from position, configuration, or internal structure. Dropping a ball converts gravitational potential into kinetic energy as the ball accelerates downward. Roller coaster designers carefully manage these conversions so that riders experience thrilling velocity shifts yet remain safe throughout the ride.
Conservation of Energy
Systems always balance their energy budgets, with no net gain or loss, but transformations occur freely. Photovoltaic panels convert sunlight into electricity, releasing minimal wasted heat if carefully designed. Firewood burning transforms chemical potential into heat and light, with the leftover ash containing some unreacted minerals. By analyzing energy flow, engineers refine processes to limit waste and bolster performance, an approach relevant to nearly every sector from manufacturing to transportation.
Power Generation and Energy Efficiency
Power quantifies how quickly energy transfers or transforms. Traditional power plants burn fuels like coal or natural gas to boil water into steam, spinning turbines that drive electrical generators. Nuclear reactors harness nuclear fission to create the same result. Renewable sources—wind, solar, hydropower—bypass fossil fuels by drawing on environmental flows. Maximizing efficiency remains crucial to meet demand while minimizing environmental strain.
Renewable Energy Sources
Wind turbines capture kinetic energy from moving air, rotating blades linked to electrical generators. Solar cells rely on semiconductor junctions that release electrons when struck by photons. Hydroelectric dams funnel flowing water through turbines, converting gravitational potential to rotational energy. Engineers and scientists devote effort to refining these methods, seeking robust power grids that integrate intermittent sources while preserving reliability. The interplay of fluid mechanics, thermodynamics, and electromagnetism underpins these advances, highlighting how fundamental physics fuels new horizons in sustainable power.
Wrapping It Up
Physics extends a unified framework that deciphers motion, energy, waves, and the forces that tie objects together, from atoms to galaxies. Newton’s laws reveal how cars accelerate and projectiles soar, while thermodynamics delineates the flow of heat that shapes climate systems and mechanical operations. Electricity and magnetism link everything from data networks to medical imaging devices, and wave phenomena clarify both the clarity of an opera performance and the workings of a fiber-optic line. Modern physics ventures into the extremes, exploring near-light-speed collisions and quantum effects that upend classical intuition.
Together, these core theories illuminate the building blocks that power every sphere of human endeavor. Ambitious engineering projects, such as constructing safer skyscrapers or sending probes to other planets, depend on precise application of gravitational equations, fluid dynamics, and advanced materials science. Emerging realms like quantum computing or nuclear fusion research highlight the ongoing promise of deeper insights into subatomic processes. Even the most seemingly routine tasks—tuning an electric guitar, steering a boat, or adjusting a house thermostat—draw unknowingly on centuries of physics discoveries.
The overarching takeaway remains that physics is not a remote set of abstractions. Instead, it is an evolving conversation about reality that bridges theoretical constructs with practical achievements. The deeper the understanding of momentum, electromagnetic waves, or thermodynamic cycles, the more effectively society can craft solutions and interpret nature’s most intriguing puzzles. By continuing to refine these principles, humanity moves closer to harnessing the workings of the universe in ways that benefit technology, sustainability, and the collective pursuit of knowledge.