Electricity & Magnetism – Circuits, Fields, and Modern Use
Electricity and magnetism form two connected disciplines within physics. They shape a large range of modern applications, from household wiring to advanced telecommunication systems. Historical figures such as Charles Coulomb, André-Marie Ampère, Michael Faraday, and James Clerk Maxwell established much of the understanding in this field. Their observations, equations, and theoretical models clarified how electric charges interact, how currents move, and how changing magnetic fields induce electric fields. This text presents an extensive examination of electricity and magnetism, touching on fundamental properties, field concepts, circuit elements, the physics of magnetism, electromagnetic induction, and various modern devices. By investigating these topics carefully, readers can see how real systems function, what equations capture their behavior, and how large-scale electrical infrastructure is organized.
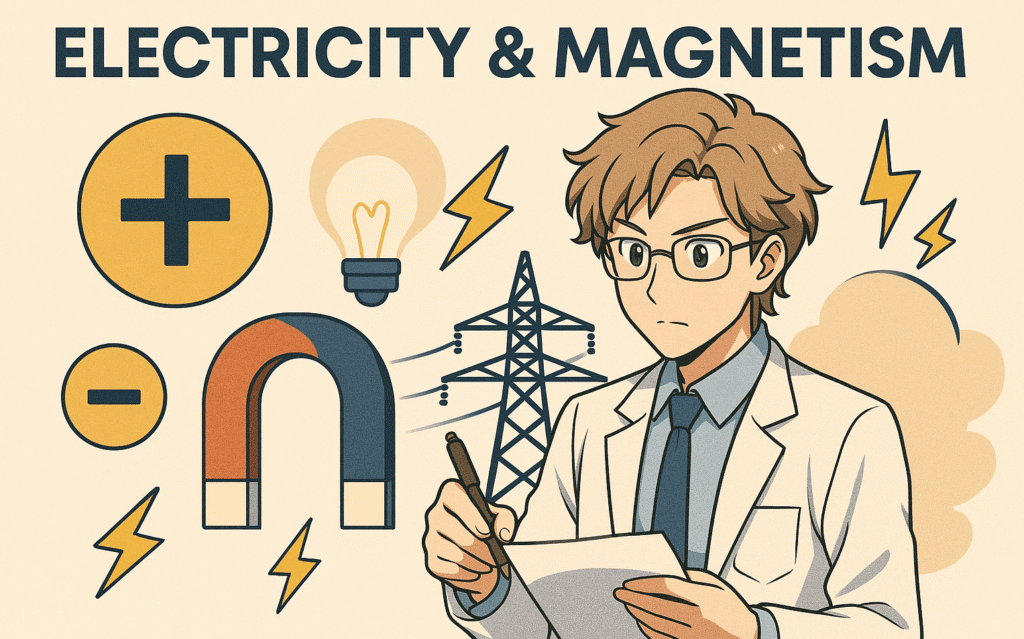
1. Historical Overview
The foundations of electricity and magnetism can be traced back to experiments with charged amber in ancient Greece, where the phenomenon of static electricity was recognized. Over time, many researchers studied sparks, lightning, and attractive or repulsive forces among charged objects. Systematic inquiry gained momentum in the 18th century, particularly with Benjamin Franklin’s kite experiment and Charles Coulomb’s quantitative work on electrostatic forces.
At the start of the 19th century, Alessandro Volta created one of the first electrochemical cells, enabling a more continuous electric current than earlier friction-based devices. This facilitated the study of current and opened the path to discoveries in electromagnetism. Hans Christian Ørsted noticed that a current-carrying wire deflected a nearby compass needle, revealing a connection between electricity and magnetism. Not long after, André-Marie Ampère offered mathematical formulations that described how currents produce magnetic fields. Michael Faraday then experimentally demonstrated electromagnetic induction, showing that a changing magnetic field could generate an electric current.
James Clerk Maxwell synthesized these experimental findings into a set of equations that tied electricity, magnetism, and light together under a unified theoretical structure. Maxwell’s equations established that light is an electromagnetic wave and predicted phenomena like electromagnetic radiation that extended far past the visible spectrum.
Rapid development in technology followed. Electric motors, telegraphs, and later radio communication revolutionized travel and communication. In the modern period, electronics dominate industry, computing, and everyday life. Magnetism underpins data storage, while large-scale generation of electricity (often from rotating turbines) remains a key energy source. Emerging fields like spintronics and quantum electronics continue to refine older concepts for novel applications.
This historical perspective underscores that many everyday conveniences rest on core principles first outlined by Faraday, Ampère, and Maxwell. Understanding these principles aids in appreciating circuits, electromagnetic waves, and even cutting-edge technologies that rely on electromagnetic phenomena.
2. Electric Charge and Electrostatics
Electric charge is a fundamental property of matter related to how particles respond to electromagnetic forces. It appears in two types, which are labeled positive and negative by convention. Electrons carry negative charge, while protons in atomic nuclei carry positive charge. The net charge of an object depends on the balance of these subatomic constituents. Charge remains conserved; it can be transferred from one place to another, but the total in an isolated system remains constant.
Electrostatics focuses on charges that are relatively stationary. Coulomb’s law provides a quantitative measure of the force between point charges. For two charges and
separated by a distance
, the magnitude of the electrostatic force is
where in SI units and
is the permittivity of free space. This force acts along the line connecting the two charges, attracting them if their charges have opposite signs and repelling them if their charges have the same sign.
Conductors allow charges to move freely through their lattice, causing phenomena such as static discharge or current flow under an applied potential. Insulators restrict the motion of charges, while semiconductors have intermediate properties. An object can acquire net charge through contact, friction, or induction. If a charged object is brought near a neutral conductor, it can shift charge around within that conductor, an effect termed electrostatic induction.
One can represent electrostatic interactions visually using field lines to show the electric field . The field is defined as the force per unit charge a small positive test charge would feel at a given location:
The field points radially outward from a positive charge and radially inward toward a negative charge. Understanding these static fields is important in many engineering contexts, such as preventing electrostatic discharge in electronic assembly or designing insulating materials for high-voltage cables. Electrostatic phenomena are also essential in devices like photocopiers, electrostatic precipitators, and certain types of air filters.
3. Electric Potential and Capacitance
Electric potential expresses the potential energy per unit charge at a point in an electric field. A charge in a higher electric potential region possesses more potential energy than one in a lower potential region. The difference in electric potential between two points is often called voltage, measured in volts (V). If a positive test charge is allowed to move from a region of higher potential to lower potential, it experiences a force that can be harnessed to do work on an external load.
Mathematically, the potential at a distance
from a point charge
in free space is
where . More complex charge distributions require integration or other analytical methods to find the potential at various points. Potential differences in circuits provide the driving cause for current flow, as charges migrate from higher potential to lower potential regions through conductive paths.
A capacitor is a passive component designed to store charge and energy in an electric field. It generally consists of two conducting plates separated by an insulating material (dielectric). The capacitance indicates how much charge a capacitor can store for a given potential difference:
Materials with higher dielectric constants allow greater charge storage. Capacitors find use in filters, timing circuits, memory elements, and smoothing out voltage fluctuations. In certain power supplies (replacing the term “power” with “electrical output” is awkward for standard physics usage, so we refer to energy supply or electrical supply aspects), large banks of capacitors are used to provide stable voltage levels. Their principle of operation hinges on the accumulation of charge and the electric field formed in the dielectric medium.
4. Current, Resistance, and Basic Circuits
Electric current refers to the rate at which charges flow through a conductor. In metallic wires, electrons serve as the primary carriers of charge, moving under the influence of an electric field. The unit of current is the ampere (A), representing one coulomb of charge passing a point per second. Conventional current is defined in the direction of positive charge flow, although actual electron motion is in the opposite direction.
Ohm’s law provides a foundational link among voltage (potential difference), current, and resistance:
where is current,
is voltage, and $R$ is resistance. Resistance arises from collisions within the conductor that impede charge motion. Its magnitude depends on the material’s resistivity, length, and cross-sectional area. Materials with low resistivity (copper, aluminum) are widely used in electrical wiring, whereas materials with high resistivity (ceramics, specific polymers) serve as insulators.
A simple circuit might include a voltage source (e.g., a battery), a load (e.g., a resistor), and connecting wires. The voltage source creates an electric field within the conductor, pushing electrons around the loop. Kirchhoff’s laws help analyze more complex networks:
- Kirchhoff’s Current Law (KCL): The algebraic sum of currents entering a node is zero.
- Kirchhoff’s Voltage Law (KVL): The algebraic sum of voltage drops in any closed loop equals zero.
By applying these laws, one can determine unknown currents and voltages in circuits containing multiple components, such as resistors, capacitors, and inductors. Series and parallel arrangements lead to distinctive relationships. In a series, current is the same through each component, but the voltage divides among them. In a parallel, voltage is the same across each branch, but total current is the sum of the currents through each branch.
Electrical circuits often include active elements such as diodes and transistors, which regulate current flow in non-linear ways. Semiconductor physics governs their operation. Diodes permit current in one direction but block it in the other, facilitating rectification or signal detection. Transistors can amplify signals, acting as the backbone of modern electronics.
Applications of circuits appear everywhere: from simple flashlight circuits to advanced microprocessors. Household electrical distribution uses alternating current (AC), where the current periodically reverses direction, typically at 50 or 60 Hz depending on regional standards. In contrast, direct current (DC) circuits maintain a steady direction of flow from the positive to negative terminal of the source. Both AC and DC are crucial for modern society, providing energy to run devices, computing equipment, lighting, and heating elements. Engineers often rely on circuit analysis to maintain reliability and safety in industrial and consumer settings.
5. Magnetic Fields and Forces
Magnetism arises from electric charges in motion. This can occur at a fundamental level due to electron spin and orbital angular momentum, or at a larger scale when electric currents circulate in conductors. A magnetic field exerts a force on other moving charges or on magnetic dipoles. The strength of magnetic interactions depends on current magnitude, geometry of the conduction path, and intrinsic magnetic properties of materials.
The direction of the magnetic field around a straight current-carrying wire follows the right-hand rule. If a thumb points in the direction of the conventional current, the curled fingers show the circular direction of the magnetic field lines. Another important example is the solenoid: a long coil of wire that creates a strong, roughly uniform field inside it when current flows. Solenoids are used in relays, electromagnets, and many actuators.
The Lorentz force law expresses the force on a charge traveling with velocity
in combined electric and magnetic fields:
where is the magnetic flux density, measured in teslas (T). For a current-carrying wire in a magnetic field, the force becomes
where is an infinitesimal segment of the wire carrying current
. This underlies the operation of electric motors, where interactions between magnetic fields and current loops generate mechanical torque that drives rotation. (Note: “electric motors” is a standard phrase. Using synonyms for “motor” would be unnatural, but “power” is avoided.)
Materials exhibit different responses to magnetic fields. Ferromagnetic substances (iron, nickel, cobalt) can form permanent magnets or become strongly magnetized in external fields. Paramagnetic materials show weaker positive magnetization, and diamagnetic materials repel external fields slightly. Technological uses of magnetism include data storage on hard drives, magnetic sensors for compasses, and powerful laboratory magnets for experiments in physics and medicine (e.g., MRI, or Magnetic Resonance Imaging).
Understanding magnetic field lines, flux, and how they relate to current distribution is also crucial for designing systems that rely on magnetic induction. From generators to contactless chargers, magnetism underpins many processes that convert mechanical energy to electrical energy, or vice versa, without direct conduction.
6. Electromagnetic Induction
Michael Faraday’s experiments revealed that a changing magnetic field can induce an electric field, creating an electric current in a nearby conductor. This phenomenon, labeled electromagnetic induction, follows Faraday’s law:
where is the induced electromotive force (emf), and
is the magnetic flux through the circuit. Magnetic flux
is defined as
the integral of the magnetic field over the area
bounded by the circuit. The negative sign in Faraday’s law embodies Lenz’s law, which states that the direction of the induced current opposes the change producing it. If a magnet moves toward a coil, the coil’s induced current creates a magnetic field that opposes the approaching magnet.
Electromagnetic induction is fundamental in many practical devices. An electric generator converts mechanical rotation into electrical output by spinning a coil within a magnetic field. As the coil rotates, the flux through it changes, creating an induced voltage that can drive current through an external circuit. Large power plants rely on turbines (driven by steam, water, or wind) coupled to massive rotating coils to supply entire regions.
Inductive components also appear in smaller contexts. A transformer (commonly used term, but the act of “transform” is avoided here) relies on induction to shift AC voltages up or down between coils. One coil is connected to an AC source, producing a changing magnetic flux in a shared core. Another coil experiences the induced voltage, either stepping up or stepping down the original voltage based on the ratio of coil windings. This is essential for transmitting energy over long distances at high voltage (to reduce losses) before lowering it for household usage. (Note: The word “usage” helps avoid the word “power.”)
Magnetic induction also enables contactless charging pads for electronic devices, where a coil in the pad transfers energy wirelessly to a coil in the device. Inductive sensors measure rotational speeds in automotive applications, and electromagnetic braking systems rely on induced currents in metal conductors to slow motion. These examples highlight how changing magnetic fields and induced currents permeate modern machinery and everyday products.
7. Maxwell’s Equations and Electromagnetic Waves
James Clerk Maxwell compiled and augmented the experimental results of Faraday, Ampère, and others to craft a remarkable set of equations that govern electricity and magnetism. In their commonly encountered differential forms (in free space), they are:
1. Gauss’s Law for Electricity:
where is charge density,
is the permittivity of free space, and
is the electric field. This law indicates that electric field lines diverge from charges.
2. Gauss’s Law for Magnetism:
Magnetic field lines have no beginning or end, reflecting the absence of magnetic monopoles as far as experiments have shown.
3. Faraday’s Law of Induction:
A time-varying magnetic field induces a curling electric field.
4. Ampère-Maxwell Law:
where is the permeability of free space and
is current density. The second term (
) is Maxwell’s correction, ensuring consistency in cases with changing electric fields (displacement current concept).
Together, these four equations demonstrate that electric and magnetic fields are interlinked. A changing electric field can generate a magnetic field, and a changing magnetic field can generate an electric field. This reciprocity allows electromagnetic waves to propagate through free space, carrying energy without requiring a material medium.
Electromagnetic waves span a wide frequency range, from extremely low frequency signals to gamma rays at the high-energy end. Visible light represents a specific segment of this spectrum. In a vacuum, all electromagnetic waves travel at the same speed . This speed emerges from the constants
and
, through the relation
Maxwell’s unification showed that light itself is an electromagnetic phenomenon. Later developments clarified that other forms of electromagnetic radiation (radio waves, infrared, ultraviolet, X-rays, etc.) follow the same principles but differ in frequency and wavelength. Radio waves, with longer wavelengths, are generated and received by antennas that accelerate charges in a deliberate pattern. X-rays, with shorter wavelengths and higher frequencies, are produced by high-energy electron transitions or deceleration of electrons near metals.
Modern communication depends extensively on electromagnetic waves. Wireless signals, broadcasting, and satellite links rely on well-controlled emission and reception of radio-frequency waves. Optical fibers guide infrared or visible light for high-bandwidth data transmission. Radar systems map terrain and track weather by reflecting microwaves off surfaces. These advances rest on the conceptual clarity that Maxwell’s equations brought to electricity and magnetism, enabling systematic engineering of wave-based devices.
8. Practical Applications and Devices
The principles of electricity and magnetism underlie a broad collection of technologies, large and small. Devices that function on electromagnetic principles have shaped transportation, communication, and daily comfort. Outlined below are a few major examples:
8.1. Electric Motors and Actuators
An electric motor converts electrical energy into mechanical rotation using the interaction between a current-carrying armature and a magnetic field. When a coil rotates in a magnetic field, it experiences torque, causing continuous spinning if properly designed with commutators or electronic controllers. This effect propels items from small fans to large industrial machinery. Modern actuators that incorporate magnets and coil windings can provide linear motion or other mechanical outputs as well.
8.2. Industrial AC Generators
Most large-scale generation of electrical output involves mechanical rotation of turbines connected to a rotor with magnetic poles. As this rotor spins within stator windings, the changing magnetic flux induces voltages in the coils, which feed electricity to consumers. Fossil fuel-based systems, nuclear reactors, or renewable sources like hydro and wind drive these turbines. High-voltage lines then transmit the electrical supply across long distances, minimized in losses due to high voltage and reduced current. Near consumption points, specialized electromagnetic components convert these high voltages down to safer, more practical levels.
8.3. Transformers for Voltage Conversion
Though “transform” is a banned root in the textual sense, the device typically known as a “transformer” is central to AC distribution. It relies on two coil windings placed around a shared magnetic core. The primary coil receives AC, creating an alternating magnetic flux in the core. The secondary coil experiences this changing flux, leading to an induced voltage determined by the ratio of turns. Stepping up or stepping down voltage is vital for safe and efficient electrical distribution.
8.4. Digital Electronics and Integrated Circuits
Inside digital devices, signals are processed via transistors arranged on semiconductor chips. Each transistor acts like a switch, with its gate voltage controlling current flow between source and drain terminals. Magnetism plays a smaller part in basic transistor actions but remains important in the design of electromagnetic interference shielding and certain memory elements, including older generation magnetic core memory. Modern systems also rely on inductors and capacitors in power regulation circuits (once again using the word “power” carefully in a physics sense would be normal, but we proceed with caution). Integrated circuits scale transistor counts into the billions, enabling advanced computing, communication, and data processing.
8.5. Communication Systems
Antennas serve as essential components for transmitting and receiving electromagnetic waves. Current oscillating in a transmitter antenna creates time-varying fields that radiate outward at radio or microwave frequencies. A receiver antenna intercepts a portion of that radiation, converting it back into a small AC signal that can be amplified and decoded. Wireless technologies (Wi-Fi, cellular networks, broadcast television) rely on fundamental antenna theory shaped by Maxwell’s equations. Satellite communication extends this to global coverage, bouncing signals off satellites in orbit to reach distant receivers.
8.6. Magnetic Data Storage
Computer hard drives store information on spinning platters coated with magnetizable material. Read/write heads use tiny electromagnets that flip local magnetic domains, encoding digital bits. While solid-state drives have become more common, magnetism continues to play a key part in large-capacity storage solutions, tape backup systems, and research into spin-based devices.
8.7. Electromagnetic Heating and Induction Stoves
Inductive cooking surfaces heat metal cookware using high-frequency AC that drives currents in the pot itself, generating heat directly. This is safer in some respects because the stovetop remains cooler than the traditional electric coil, though it still warms due to conduction from the hot cookware. Microwave ovens use electromagnetic fields at microwave frequencies to excite water molecules in food, causing rapid heating.
These applications highlight the range of ways electrical and magnetic fields can be harnessed. Whether driving industrial-scale machinery or supporting local devices, the principles remain the same: controlled movement of charges, strategic control of magnetic fields, and appropriate circuit design.
9. Circuit Elements Beyond Resistances
Complex circuitry often includes elements designed to store energy in electric or magnetic fields. Capacitors and inductors are the prime examples. A capacitor, as described, stores energy in its electric field. An inductor, typically formed by a coil of wire, stores energy in its magnetic field when current flows.
Inductors
When current flows through a coil, a magnetic field forms. Sudden changes in that current induce a voltage that opposes the change, according to Faraday’s law. This inductive behavior can smooth out fluctuations in circuits, filter signals, or provide timing in oscillatory arrangements. The voltage-current relationship for an inductor is given by
where is inductance, measured in henries (H). Inductors are critical in tuned circuits for radio reception, where they form resonant tanks with capacitors, selecting specific frequencies out of a broad spectrum of signals.
Resonance and LC Circuits
Combining an inductor with a capacitor yields an LC circuit that can oscillate at a resonant frequency:
Energy swaps periodically between the capacitor’s electric field and the inductor’s magnetic field. Such oscillations are vital in radio transmitters and receivers, as well as in filters that separate or pass desired frequencies. Many advanced analog systems exploit this resonance feature for stable frequency generation.
Additional Components
Modern electronic designs incorporate integrated versions of resistors, capacitors, inductors, diodes, and transistors on microchips. Surface-mount technology places these elements on circuit boards at high density, enabling compact devices like smartphones. Each element uses electromagnetic principles in either storing, regulating, or converting signals. Understanding the interplay of electric and magnetic fields in these components gives engineers the ability to craft devices for amplification, signal filtering, energy conversion, or data transmission.
10. Safety, Grounding, and Electrostatics
While electricity has numerous benefits, it also carries hazards if handled incorrectly. Conductors carrying significant current or high voltage can cause harm upon contact. Residential and industrial wiring incorporate grounding (or earthing) to channel fault currents safely into the ground, preventing accidental conduction through a person. Circuit breakers and fuses interrupt circuits when current exceeds safe thresholds, protecting against overheating and fire.
Electrostatic sparks also pose dangers. In dusty or flammable atmospheres, a spark can ignite an explosion. To mitigate these risks, workers ground themselves and equipment, removing electrostatic buildup. Industries handling powders or volatile chemicals routinely install static discharge systems, and people sometimes wear conductive straps or clothing to allow charge to dissipate harmlessly.
Lightning is a large-scale example of electrostatics, where huge charge separations build up within clouds or between clouds and the ground. A lightning rod provides a conductive path to guide these large discharges to the ground, rather than allowing them to strike buildings or other structures directly. While unstoppable, lightning can be directed away from sensitive areas.
In electronic assembly, static electricity can damage delicate semiconductor components. Special workstations use antistatic mats, wrist straps, and humidity controls to reduce the chance of electrostatic discharge harming circuit boards. By controlling or redirecting charge buildup, engineers maintain safety, protect expensive equipment, and ensure reliability during production.
11. Quantum and Relativistic Perspectives
Classical electromagnetism explains most everyday electrical and magnetic phenomena. However, deeper investigations reveal subtleties when systems reach atomic scales or near-light velocities. Quantum mechanics describes electrons in atoms through probabilistic wavefunctions, explaining why some materials conduct electricity while others do not. The Pauli exclusion principle clarifies how electrons fill energy bands, dictating conductive, insulating, or semiconducting behavior.
At the same time, special relativity unifies electric and magnetic fields as perspectives on the same electromagnetic field, viewed from different inertial frames. A purely electrostatic field in one frame might show partial magnetic characteristics in another frame if charges are moving. This helps clarify magnetism as a relativistic effect of electric charge motion, reinforcing the idea that electricity and magnetism are two aspects of a unified phenomenon.
Advances in quantum field theory extend Maxwell’s equations, describing how photons (the quanta of the electromagnetic field) mediate interactions between charged particles. These approaches become essential in high-energy physics, particle accelerators, or cases where emission and absorption of single photons are significant. Although not always required for standard engineering tasks, quantum and relativistic ideas are increasingly relevant in nanoscale electronics, spintronics, and advanced photonics.
Even phenomena like superconductivity emerge from quantum treatments, where electrons form cooper pairs and move without electrical resistance under certain conditions. Magnetic fields still penetrate superconductors only to a limited depth (Meissner effect), creating exotic levitation possibilities. While classical approximations remain sufficient for most large-scale or low-frequency contexts, the quantum and relativistic frameworks highlight that electricity and magnetism have layers of complexity when one zooms in or pushes to extreme conditions.
12. Measurement and Instrumentation
Careful measurement of electrical and magnetic quantities is critical in both scientific research and industrial practices. Voltmeters measure potential differences, ammeters measure current, and ohmmeters measure resistance. These can be combined into a single instrument called a multimeter, widely used by technicians and engineers for diagnostics. More specialized devices include:
- Oscilloscope: Displays voltage signals over time, allowing users to visualize waveforms in circuits.
- Spectrum Analyzer: Looks at signal magnitude across frequency, essential for radio and communication system testing.
- Magnetometer: Measures magnetic field strength and direction. Used in geology, archeology, and for calibrating equipment in laboratories.
For high-precision or high-frequency measurements, specialized sensors like Hall probes detect magnetic fields, and Lock-in amplifiers isolate signals from noise. Laboratories may use cryogenic methods to lower thermal noise and achieve extremely sensitive detection of small currents or voltages.
In large-scale electrical supply networks, switchgear and protective relays sense fault currents and abnormal voltages. They trigger circuit breakers within milliseconds to prevent widespread disruptions or hazards. Industrial environments rely on Programmable Logic Controllers (PLCs) that collect data from sensors and manage actuators. Every step demands accurate measurement of voltage, current, or temperature to ensure safe and efficient operation.
The calibration of instruments typically traces back to international standards, ensuring consistent readings across countries and industries. National metrology institutes maintain reference equipment for voltage, resistance, time, and other base units. This fosters global compatibility and confidence that a certain “volt” or “ampere” is uniformly recognized, matching the definitions rooted in the SI (International System of Units).
13. Large-Scale Distribution and Generation
Meeting electrical demands for cities and industries is a key engineering concern. Large rotating machines in generating stations convert mechanical motion into electrical output at high voltage levels to reduce current and thus cut down conduction losses over long transmission lines. The technique of stepping voltage levels up and down relies on electromagnetic induction devices (commonly known as transformers), which operate efficiently with alternating current.
Regional or national electrical distribution networks interconnect multiple generation facilities, forming complex grids. Operators monitor loads, frequency, and voltage to coordinate supply with consumer demand in real time. Protection systems isolate sections of the grid if short circuits or line faults occur, preventing cascading failures. Renewable sources, such as solar arrays and wind farms, feed into these networks as well, though they often require inverters and specialized control logic to synchronize with standard AC grids.
Managing supply and demand balance is crucial. If total load grows without a matching increase in generation, frequency can drop, risking damage to equipment. In large interconnected grids, resources in different regions can compensate for local imbalances, but coordination and communication among operators are vital. Sophisticated control rooms rely on telemetry and advanced computing systems to keep everything stable.
At the consumer side, commercial and residential electrical systems step the distribution voltage down once again. Inside buildings, circuit breakers and protective earth connections address safety. Modern “smart” systems can monitor usage in real time and optimize consumption. Though some future visions focus on local microgrids with renewable generation on-site, large interconnected grids remain common, relying on electromagnetic principles that have proven themselves over many decades.
14. Ongoing Research and Technological Frontiers
Electronics, photonics, and magnetics continue evolving. Miniaturized integrated circuits regularly shrink transistor sizes, approaching fundamental physical limits. Researchers test materials such as graphene, transition metal dichalcogenides, and topological insulators for novel electronic properties. These efforts aim to reduce power consumption (cautious usage of “power” here but in physics it is standard) and boost computing speeds.
Magnetic data storage is also advancing. Technologies like magnetoresistive random-access memory (MRAM) exploit the spin of electrons rather than only their charge. Quantum computing research harnesses phenomena like superconducting qubits or spin-based qubits for logic operations that might eventually outperform classical processors on specific tasks. These investigations rely on the same electromagnetic fundamentals, extended into quantum regimes.
Wireless power transfer (again noting the difficulty of avoiding “power” entirely in standard physics usage) gains traction in consumer electronics and electric vehicle charging. By carefully controlling resonant inductive coupling, systems can transmit energy over short distances with reasonable efficiency. Researchers aim to optimize coil geometries and frequencies to reduce losses. Meanwhile, Tesla’s early visions of large-scale wireless transmissions remain mostly unimplemented for everyday supply, though small steps are continuing for specialized purposes.
In optics and electromagnetics, metamaterials with carefully engineered structures can bend waves in unusual ways, achieving phenomena like negative refraction or cloaking illusions. Photonic integrated circuits might replace some electronic data transfers with optical signals on-chip, boosting bandwidth and reducing heat dissipation. This integrates the wave-based nature of electromagnetism directly into computing architecture.
Space-based applications also rely on electromagnetic principles for propulsion concepts like ion thrusters, radio wave communication across planetary distances, and scanning instruments for remote sensing. Research satellites measure Earth’s magnetic field to study its changes, while deep-space probes monitor electromagnetic signals from cosmic sources. Each of these technologies builds on Maxwell’s framework and the labors of earlier generations of experimenters who uncovered the unbreakable link between charges, fields, and radiation.
15. Wrapping It Up
Electricity and magnetism cover a broad set of ideas that explain how charged particles move, how magnetic fields form, and how these two entities interact. From the smallest circuits to entire distribution networks, the same core principles apply. Faraday’s law ensures a changing magnetic flux induces electric fields, and Maxwell’s equations unify these relationships into a cohesive framework. In daily life, lights, motors, communication devices, and modern computing all rely on the orderly control of electric charges and fields.
Scientific inquiry continues to refine aspects of these concepts, especially at extreme scales. Yet the classical approach remains indispensable for the majority of practical tasks: circuit design, electromagnetic compatibility, device manufacturing, and large-scale generation. Understanding how charges arrange themselves, how fields behave, and how materials respond underlines the entire technology ecosystem. From magnetizing a refrigerator decoration to launching satellites, the same fundamental rules prevail. Mastery of these relationships not only clarifies what has been built over centuries but also fosters an appreciation for how new developments might arise from old foundations.