Analytical Chemistry – Methods, Tools & Real-World Uses
Analytical chemistry focuses on examining matter to determine its composition, structure, and quantity of constituents. Professionals in this field employ methods designed to separate, identify, and quantify chemical species in samples. It underpins activities across science, medicine, forensics, quality control, and more. This guide explores central ideas in analytical chemistry, discussing classic and modern methods, instruments, and the considerations that ensure accurate and reliable results.
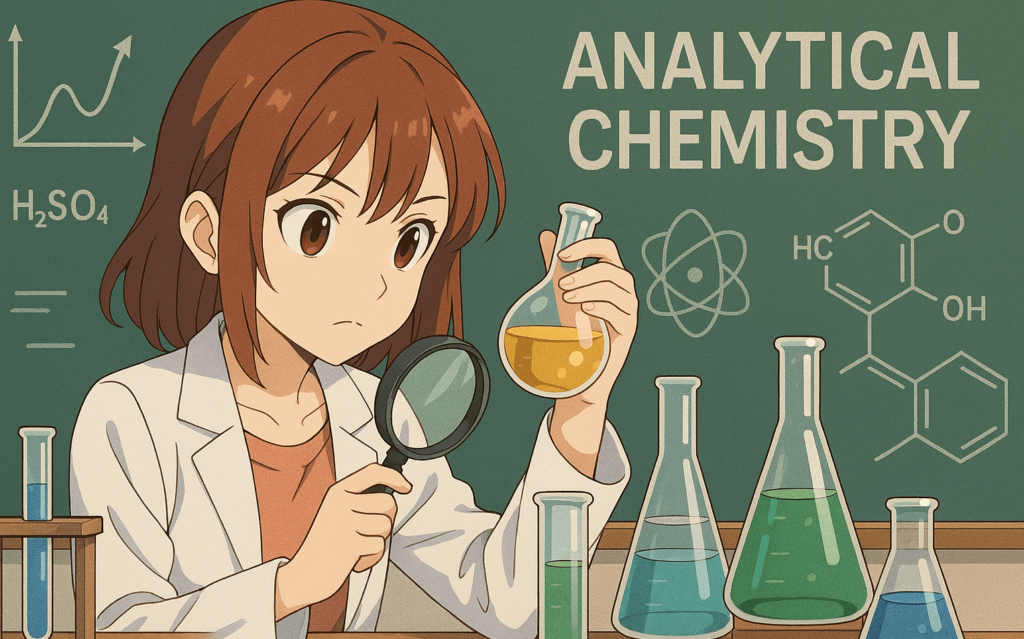
1. Overview of Analytical Chemistry
Analytical chemistry supports understanding of materials, from trace contaminants in water to active pharmaceutical ingredients in medications. Its methods enable laboratories to uncover what elements or compounds exist, measure concentrations, and track changes over time or under different conditions.
Applications are vast. Environmental labs rely on these techniques to gauge pollutants, while clinicians employ them to measure hormones or drugs in blood. Food producers confirm product safety and consistency through routine checks. Research scientists test new compounds or monitor reaction outcomes. In each scenario, analytical methods must deliver precise and reliable data.
2. Qualitative vs. Quantitative Analysis
2.1. Qualitative Analysis
Qualitative analysis addresses whether a particular substance is present. Classic examples include colorimetric reactions that reveal certain ions (like chloride giving a white precipitate with silver nitrate). Advanced instrumental methods, such as mass spectrometry, can identify compounds by fragmentation patterns, while infrared spectroscopy highlights functional groups.
- Precipitation Tests: A known reagent forms an insoluble solid if a target ion exists.
- Color Indicators: Some tests rely on visible color changes (e.g., litmus paper for acidity).
- Spectral Signatures: Distinct absorption or emission spectra confirm certain elements or molecules.
2.2. Quantitative Analysis
Quantitative analysis measures how much of each substance exists. Gravimetric methods rely on weighing a pure compound formed from the analyte. Titrations measure volume of a reagent needed to react fully with the analyte. Modern labs frequently use calibration curves from spectroscopy or chromatography instruments.
- Titration: An acid-base titration can quantify the amount of acid in a sample. Redox titrations measure electron transfer, while precipitation titrations can confirm halides or other ions.
- Gravimetric Analysis: The analyte is converted into a product of known composition, then dried and weighed.
- Instrumental Quantification: Concentrations can be inferred by comparing signals (peak area, absorbance) to standards at known levels.
3. Classical Analytical Methods
3.1. Gravimetry
In gravimetric approaches, the analyte is transformed into a stable, pure compound, which is then isolated and weighed. For instance, a water sample containing sulfate might be precipitated as barium sulfate, filtered, dried, and weighed. From the mass of barium sulfate, one can calculate the original sulfate content. Although time-consuming, gravimetry often yields high accuracy because the measurement depends only on mass, which can be precisely determined.
3.2. Volumetry (Titrations)
Volumetric analysis uses solutions of known concentration (standard solutions) to determine unknown quantities in a sample. The end point is observed by a color change or an instrumental signal (potentiometric, photometric). Titrations fall into categories, such as:
- Acid-Base Titrations: Involving neutralization reactions, typically guided by pH indicators.
- Redox Titrations: Oxidizing or reducing agents measure the electron-transfer capacity.
- Complexometric Titrations: A chelating agent (EDTA) complexes with metal ions.
Accuracy hinges on correct standardization of titrant and clear detection of the endpoint. Burettes, pipettes, and well-chosen indicators ensure repeatable data.
3.3. Precipitation Reactions
Precipitation-based methods can identify or quantify an ion by forming an insoluble salt. An example is the chloride analysis via silver nitrate, where a white precipitate of silver chloride forms. Interference arises if other ions also form precipitates. Nonetheless, these methods remain foundational in many teaching labs, providing straightforward demonstrations of stoichiometry and ionic reactions.
4. Sample Preparation
Before analysis, samples must often be collected and processed with care. The goal is to yield a representative specimen free from contamination and in a compatible form for testing. Techniques vary:
- Filtration or Centrifugation: Removes particulates before analyzing a liquid.
- Digestion: Breaks down solid matrices (soils, tissues) using acids, microwaves, or fusion with fluxes, releasing target elements into solution.
- Extraction: Solvent extraction pulls out desired compounds from complex mixtures (e.g., separating pesticides from food). Solid-phase extraction uses cartridges that trap analytes on adsorbent resins.
- Derivatization: Chemically modifies certain groups to improve detection or separation, particularly in chromatography (e.g., silylation of polar groups for GC analysis).
Proper sample handling also involves stable storage, correct labeling, and chain-of-custody procedures, ensuring data validity and traceability.
5. Separation Techniques
5.1. Chromatography
Chromatographic methods separate mixtures based on differential interactions with a stationary phase and a moving phase. Variations exist for gases, liquids, or supercritical fluids:
- Gas Chromatography (GC): Volatile samples pass through a capillary column coated with a stationary phase, with temperature programming often employed. Detectors range from flame ionization (FID) to mass spectrometry (GC-MS).
- High-Performance Liquid Chromatography (HPLC): Uses high pressure to push solvent and dissolved analytes through a packed column. Reverse-phase HPLC (with a nonpolar stationary phase) is common for pharmaceuticals or biomolecules. UV-Vis detectors or mass spectrometers (LC-MS) measure eluate composition.
- Ion Chromatography: Separates ions (like anions or cations) using ion-exchange resins, crucial for environmental and food testing.
Chromatography provides both qualitative (peak retention times) and quantitative (peak areas or heights) data. Correct calibration, column care, and method optimization are essential.
5.2. Electrophoresis
Electrophoresis uses an electric field to move charged analytes through a medium (gel, capillary). Molecules separate by size or charge:
- Gel Electrophoresis: Agarose or polyacrylamide gels for DNA or proteins. Bands reveal approximate molecular weight or presence of specific fragments.
- Capillary Electrophoresis: High-voltage separation in narrow capillaries. Offers high resolving power, minimal sample use, and short run times. Linking capillary electrophoresis to mass spectrometry (CE-MS) enhances detection specificity.
Electrophoresis underpins genetic research, protein characterization, and many clinical tests.
6. Spectroscopic Techniques
6.1. UV-Vis Spectroscopy
Ultraviolet-visible spectrophotometry measures absorption of light in the UV to visible range, reflecting electron transitions in molecules or ions. Beer’s Law relates absorbance to concentration. Analysts employ standard curves or direct absorptivity constants to quantify analytes like transition metal complexes or organic chromophores.
- Applications: Checking drug purity, measuring nitrate in water, monitoring reaction kinetics.
- Strengths: Simple instruments, quick operation.
- Limitations: Interferences if overlapping absorbance or turbid samples appear.
6.2. Infrared (IR) Spectroscopy
IR spectroscopy probes vibrational modes of molecules. Each functional group has characteristic absorption bands (e.g., broad O–H peak around 3200–3600 cm⁻¹). Attenuated total reflectance (ATR) accessories permit direct solid or liquid sample analysis with minimal preparation.
- Applications: Identifying unknown solids or liquids, verifying polymer composition, checking functional group changes.
- Fourier Transform IR (FTIR) instruments provide rapid scans and strong signal-to-noise ratios.
6.3. Atomic Spectroscopy
Atomic absorption (AA), atomic emission (AES), and inductively coupled plasma (ICP) methods measure metals and select nonmetals:
- Flame AA: The sample is atomized in a flame. A lamp of the target element’s emission line passes through the flame, and the analyte absorbs at that wavelength.
- ICP-OES: Inductively coupled plasma (ICP) excites atoms; the emission at specific wavelengths reveals their concentration. Suited for multi-element analysis with high sensitivity.
- ICP-MS: ICP ionizes the sample, and a mass spectrometer separates ions by mass-to-charge ratio. Exceptional sensitivity allows detection of trace metals at parts-per-trillion levels.
Atomic techniques are crucial in environmental labs (tracking lead, cadmium in water), food safety (monitoring arsenic in rice), or industrial quality control (checking metals in alloys).
6.4. Nuclear Magnetic Resonance (NMR) Spectroscopy
NMR examines nuclear spin states (commonly ¹H or ¹³C). A strong magnetic field and radiofrequency pulses yield spectra that reflect the electronic environment around nuclei. Peak positions (chemical shifts) and splitting patterns convey structural details:
- ¹H NMR: Indicates hydrogen environments.
- ¹³C NMR: Explores carbon skeletons.
- Advanced Techniques: DEPT, HSQC, HMBC reveal connectivity and substituent patterns.
NMR is indispensable for organic structure elucidation, conformational studies, and purity checks. It can also quantify mixtures if integrated carefully.
6.5. Mass Spectrometry (MS)
Mass spectrometers ionize molecules, measure their mass-to-charge (m/z) ratios, and often fragment them to produce characteristic patterns. Coupling MS to GC, LC, or CE offers powerful separation plus identification.
- Electron Ionization (EI): Common in GC-MS for small molecules. Produces a typical fragmentation pattern.
- Electrospray Ionization (ESI): Soft ionization for larger biomolecules, widely used in LC-MS.
- Matrix-Assisted Laser Desorption Ionization (MALDI): Suited for proteins, peptides, polymers, with time-of-flight (TOF) mass analyzers giving mass fingerprints.
MS data confirm molecular weights, structures, and trace impurities, often critical in pharmaceutical or forensic labs.
7. Electroanalytical Methods
7.1. Potentiometry
Potentiometric measurements track voltage differences between two electrodes. The classic example is the pH meter, using a glass electrode for hydrogen ion activity. Ion-selective electrodes detect specific ions (like fluoride or potassium) by selective membranes, supporting environmental or clinical tests.
7.2. Voltammetry and Amperometry
In voltammetry, current is measured while varying the applied potential at an electrode. Analytes oxidize or reduce, producing characteristic waves or peaks. Techniques include polarography, cyclic voltammetry, and differential pulse voltammetry. Amperometry measures current at a fixed potential (e.g., glucose sensors in medical diagnostics). These methods excel at analyzing electroactive species, sometimes down to micromolar or nanomolar concentrations.
7.3. Coulometry
Coulometric assays involve measuring total charge passed during complete electrolysis of the analyte. Faraday’s laws relate charge to moles of substance reacting. Though less common, coulometry can be highly accurate for certain analytes, especially at small concentrations, given the stoichiometric nature of the electrochemical reaction.
8. Thermal Analysis
Thermal methods observe changes in a sample’s properties as temperature shifts:
- Thermogravimetric Analysis (TGA): Monitors mass changes during heating. Useful for decomposition behavior, moisture content, or residue analysis.
- Differential Scanning Calorimetry (DSC): Measures heat flow differences to or from a sample versus a reference. Identifies melting points, crystallization, or phase transitions.
- Differential Thermal Analysis (DTA): Tracks temperature differences under controlled heating.
These approaches characterize polymers, pharmaceuticals, or other materials, revealing stability or composition details.
9. Data Handling and Quality Assurance
9.1. Calibration and Standardization
Reliable measurements require calibration with known references. Multi-point calibrations form a linear or nonlinear curve. Quality control samples verify that instruments remain accurate over time. Internal standards compensate for losses or matrix effects in complex samples.
9.2. Method Validation
Valid methods must show good specificity, linearity, accuracy, precision, detection limit (LOD), and quantification limit (LOQ). Regulatory bodies often require thorough documentation for pharmaceutical or environmental analyses. Robustness checks see how small changes in temperature, pH, or reagent purity affect results.
9.3. Statistical Analysis
Error analysis, standard deviations, and confidence intervals quantify data reliability. Replicate measurements detect random variation, while method blanks reveal background contamination. Tools like ANOVA compare multiple sets of data. For complex pattern recognition, analysts may turn to chemometrics (multivariate analysis).
9.4. Laboratory Information Management
Modern labs implement software systems to track samples, manage instrument outputs, and store results. Electronic records improve traceability and reduce transcription mistakes. For critical fields, such as forensics or pharmaceuticals, secure auditing trails confirm data integrity throughout the process.
10. Advancements and Special Topics
10.1. Miniaturization and Lab-on-a-Chip
Smaller devices can perform separations or assays on microfluidic chips, using tiny sample volumes and integrated detection. This approach reduces cost, speeds testing, and enables point-of-care diagnostics. Examples include handheld blood analyzers or portable environmental sensors for field measurements.
10.2. Hyphenated Techniques
Combining multiple methods, such as GC-MS, LC-MS, or ICP-MS, merges separation power with specific detection. More advanced combos (LC-NMR-MS) or MS imaging (MALDI imaging) offer new ways to study materials or tissues. These integrated systems push the boundaries of detection limits and structural elucidation.
10.3. Chemometrics and Big Data
Sophisticated software and large data sets let scientists extract more information from each experiment. Spectral libraries, pattern recognition, and advanced algorithms can classify unknown compounds or predict properties. Machine learning further refines method development, assisting in real-time correction of instrument drifts or sample matrix effects.
11. Role in Industry and Research
11.1. Pharmaceutical Development
Analytical labs confirm that active ingredients meet purity standards, detect impurities, and evaluate stability under various conditions. Validation of each method is essential before drug approval. Advanced instruments ensure robust analysis of complex formulations, helping researchers verify bioequivalence and batch-to-batch consistency.
11.2. Environmental Monitoring
Authorities rely on analytical chemistry to enforce pollution limits, track water quality, and identify emerging contaminants. Techniques like ICP-MS or HPLC measure toxic metals or pesticide residues at extremely low levels. On-site field kits or mobile labs can test for nitrates, heavy metals, or microorganisms quickly, supporting early intervention.
11.3. Food Safety and Quality Control
Routine screenings check for adulterants, pesticide remains, or nutritional content. Chromatography and spectroscopy detect illegal dyes, antibiotic traces in meat, or mycotoxins in grains. Rapid screening ensures that products meet regulatory standards and protect consumer health.
11.4. Forensic Analysis
Crime laboratories analyze bodily fluids, gunshot residue, or trace evidence (fibers, paint chips) to link suspects or events. DNA profiling, drug identification, and toxicology all hinge on robust analytical methods. Evidence chain-of-custody practices and thorough method validation safeguard the integrity of results in legal contexts.
12. Future Outlook
Analytical chemistry continues evolving with faster, more sensitive instruments and improved data interpretation. Scientists are designing greener approaches, minimizing reagents and waste. Portable analyzers using advanced sensors or miniaturized mass spectrometers may become routine in clinics or field investigations. Artificial intelligence could streamline method development or automate result interpretation, further boosting productivity.
This progress underscores the discipline’s enduring significance, as technology and society expand demands for accurate, high-throughput analysis. From diagnosing diseases to protecting the environment, analytical chemistry is integral to ongoing efforts to manage resources, ensure safety, and fuel scientific discoveries.