Key Concepts in Chemistry
Chemistry Essentials – Concepts and Practical Examples
Chemistry stands as a discipline that deeply shapes technological advancement, healthcare progress, and our everyday environment. Each concept, from the internal structure of atoms to the global effects of chemical processes, influences how materials are produced, how medicines are formulated, and how ecosystems are preserved. The content below explores major topics in chemistry, linking theoretical foundations to practical outcomes that affect household tasks, industrial applications, and current research pursuits.
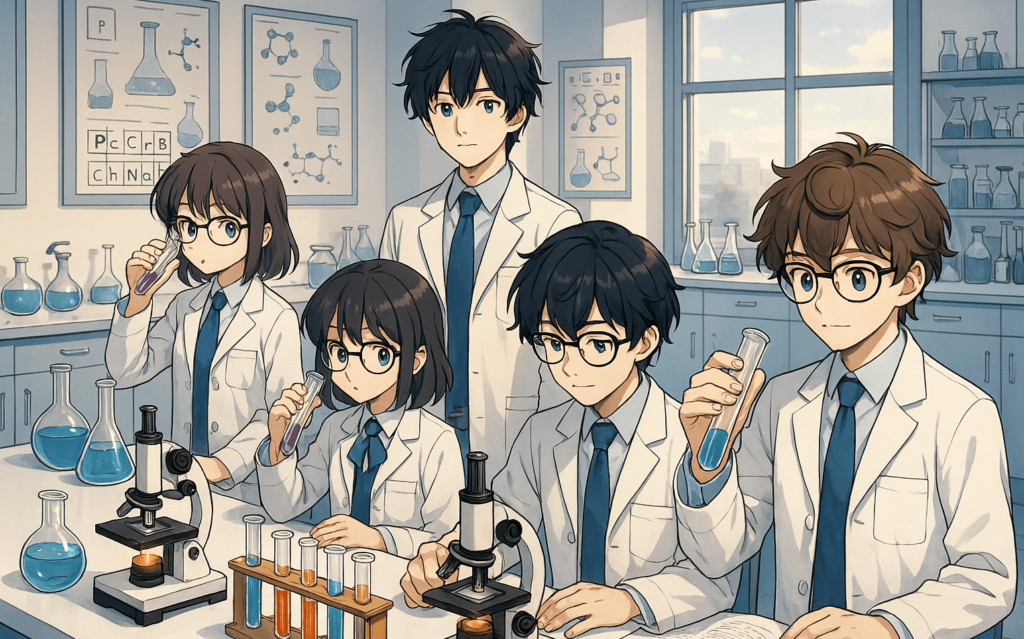
Atomic Structure
All chemical behavior begins with an understanding of atoms, those minuscule units that combine in vast quantities to form matter. Each atom contains protons and neutrons in a core called the nucleus, surrounded by electrons that occupy distinct energy levels. Variations in the electron arrangement often determine how an element reacts when combined with another. Hydrogen’s single electron, for instance, leads to different properties than carbon’s six, which in turn differs from iron’s 26.
Isotopes present an intriguing twist on the atomic theme. An element like carbon always has six protons, yet the number of neutrons can differ, forming stable or radioactive variants. Carbon-14, which undergoes radioactive decay, finds use in archaeology and geology for age determination. Elsewhere, radioactive isotopes serve in medical imaging, letting healthcare professionals trace metabolic pathways or capture detailed internal snapshots. This dual use of isotopes, spanning both research and diagnostic activities, illustrates how atomic structure underpins remarkable technologies in medicine, environmental science, and other fields.
Periodic Table
One of the greatest organizational triumphs in science, the periodic table arranges elements according to their atomic number and chemical traits. Rows, known as periods, reflect repeating patterns in electron configurations, while columns, or groups, assemble elements with similar bonding tendencies. Patterns in size (atomic radius), electron affinity, and electronegativity allow researchers to anticipate reactivity even before an element is fully explored in the lab.
Many modern breakthroughs in materials science emerged from understanding these repeating trends. Electronics rely on semiconductors like silicon and germanium, which show specific conductive properties between metals and nonmetals. That characteristic, discovered through systematic experimentation guided by periodic insights, paved the way for microprocessors, solar cells, and a range of digital devices.
Bonding, Reactions, and Transformations
Chemical reactions can only unfold when atoms or molecules form or break bonds, and the manner of bonding—ionic or covalent—directly impacts the resulting compounds’ stability and properties. Ionic bonding arises through the transfer of electrons, creating charged particles (ions) that attract each other strongly. Familiar compounds like sodium chloride (table salt) illustrate the rigid lattice typical of ionic materials. By contrast, covalent bonds entail electron sharing, producing molecules with different melting points, solubilities, or reactivities. Water, which has polar covalent bonds, dissolves a wide array of substances and shapes countless biochemical reactions.
These foundational concepts drive manufacturing processes and product development. The plastic containers in a kitchen, for instance, rely on polymer networks formed primarily by covalent bonds that link organic monomers into long chains. Meanwhile, strong ionic interactions support industrial ceramics used in high-temperature environments. By recognizing why certain compounds have notable strength or conductivity, professionals can propose improved formulations for uses in construction, electronics, and biotechnology.
Stoichiometry
Reactions do not merely depend on the presence of correct molecules; proportions matter deeply. Stoichiometry quantifies how much of each reactant is needed to form a given amount of product, ensuring processes minimize waste and meet expected yields. Scientists apply the mole concept to bridge the gap between atomic-scale interactions and macroscopic amounts. One mole corresponds to Avogadro’s number of particles (approximately 6.022×10^23), a benchmark that connects the symbolic realm of chemical equations to actual mass in grams.
This principle is indispensable in industrial facilities that mass-produce items such as pharmaceuticals, cleaning agents, or polymers. Missing or exceeding the proper stoichiometric ratio can lead to inefficiencies, higher costs, or product inconsistencies. Research and development teams refine these calculations to maintain safety, quality, and compliance. In smaller-scale scenarios, teachers demonstrate stoichiometry through classroom experiments that highlight the significance of balanced equations, guiding students toward a deeper appreciation of precise chemistry.
The Chemistry of Acids, Bases, and Solutions
Acids, bases, and their properties connect deeply with real-world experiences. Acids typically donate protons (H^+), while bases accept them, measured by the pH scale that runs from 0 (highly acidic) to 14 (strongly basic). Household items such as vinegar (acetic acid) or baking soda (a weak base) demonstrate these characteristics in cooking, cleaning, or small do-it-yourself tasks. Meanwhile, industries rely on acids and bases for large-scale processes, like refining metals or manufacturing detergents.
Solutions represent homogeneous mixtures of a solute dissolved in a solvent, providing frameworks for countless chemical processes. In medicine, saline and intravenous solutions are designed to match body fluid composition, preventing cellular damage and ensuring safe administration of electrolytes. Concentration units—molarity, molality, and mole fraction—help scientists or clinicians evaluate dosage accuracy, reactivity potential, or environmental impact. Even beverage industries apply these metrics when regulating sugar content or controlling fermentation stages.
Thermochemistry and Energy Changes
Every reaction involves energy exchange in one form or another, an aspect studied under thermochemistry. Reactions either release energy (exothermic) or consume it (endothermic), with heat transfer shaping how feasible or spontaneous a process can be. Enthalpy, entropy, and Gibbs free energy function as theoretical signposts that outline the direction and balance of these events.
In everyday life, disposable hand warmers illustrate an exothermic reaction. When iron filings and activated carbon meet oxygen, the subsequent oxidation releases heat. Instant cold packs, meanwhile, display endothermic dissolutions of chemicals like ammonium nitrate, cooling the surroundings. These items highlight how controlling reaction energy yields practical convenience. On a broader scale, thermochemical studies inform power generation methods: engineers examine which fuels or reaction pathways convert stored chemical energy into electricity most efficiently, while also analyzing potential pollutants and environmental outcomes.
Electrochemistry and Battery Innovations
Redox reactions, involving electron transfer, fuel countless devices. Galvanic cells transform chemical energy into electrical current, forming the basis of common batteries, while electrolytic cells rely on external power to drive non-spontaneous reactions. Understanding oxidation states, electrode potentials, and cell construction is key to unlocking the ways a battery delivers continuous current or recharges for repeated use.
Rechargeable lithium-ion batteries exemplify electrochemistry’s impact on daily life. Gadgets like phones, laptops, and electric cars all depend on the efficient movement of lithium ions between electrodes. Researchers refine cathode and anode materials, seeking longer run times, faster charging, and reduced safety risks. The same chemistry guides supercapacitor designs that excel in rapid energy release, essential in certain industrial applications requiring surges of power. As the global community seeks greener energy solutions, electrochemistry remains at the forefront of next-generation storage technologies.
Organic Chemistry and Real-World Materials
Although “organic” often conjures images of living creatures, organic chemistry zeroes in on carbon-based compounds and the ways they link with hydrogen, oxygen, nitrogen, or other elements. This domain governs the structure and reactions of countless molecules, from everyday plastics to the small-molecule drugs prescribed by physicians. Core features include functional groups—alcohols, carboxylic acids, amines—that dictate a compound’s behavior.
Drug discovery illustrates why organic chemistry stands so important. By testing how molecular skeletons and functional groups interact with biological targets, pharmaceutical teams can tune therapies for better efficacy or fewer side effects. Aspirin, originally derived from willow bark, provides an example: its active ingredient features an ester group that eases discomfort. Polymers also originate from organic reactions, forming everything from biodegradable packaging to specialized prosthetics for medical implants. This interplay of structure, bonding, and reactivity drives technological advancement across multiple fields.
Biochemistry and Cellular Processes
Biochemistry overlaps biology and chemistry, dissecting the molecular machinery fueling life processes. Cells rely on carbohydrates, proteins, lipids, and nucleic acids for energy, structural integrity, and genetic transmission. Enzymes, which are protein catalysts, expedite essential chemical reactions, from sugar breakdown to the replication of DNA. This synergy enables organisms to grow, repair tissue, and adapt to environmental pressures.
Healthcare breakthroughs frequently arise from unraveling these biochemical pathways. Enzyme inhibition or activation can correct dysfunctional metabolic cycles, forming the basis of treatments for conditions like high cholesterol or type 2 diabetes. Scientists also investigate the biochemistry of viruses and bacteria to outsmart antibiotic resistance. In agriculture, breeding methods or genetic tools target metabolic pathways in crops, optimizing yield, nutritional content, or pest resistance. By examining macromolecules and their transformations, researchers strengthen the foundation for knowledge about life’s complexities and how to harness them.
Environmental Chemistry and Sustainable Practices
Chemistry does not operate in a vacuum; it intersects with soil, water, and air on a planetary scale. Environmental chemistry focuses on pollutants, nutrient cycles, and how anthropogenic substances affect ecosystems. Understanding how chemicals disperse and degrade informs decisions about waste management, emissions control, and legislation designed to safeguard public health.
Green chemistry arises as a proactive endeavor in this context. Instead of dealing with pollution after it has occurred, practitioners refine processes to lower hazardous byproducts and reduce energy usage. Catalysts tailored to specific reactions help industries realize more efficient conversions with fewer toxic leftovers. Biodegradable plastics rely on chemical structures that degrade under composting conditions, easing the burden on landfills. Phytoremediation, a method of cleaning contaminated environments using specialized plants, underscores the potential of harnessing natural processes. Whether dealing with urban smog or greenhouse gases, chemists strive to create or modify chemicals in a manner that supports ecological balance.
Analytical Chemistry and Detection
Finding and quantifying substances underpins quality control, medical testing, and investigative work. Analytical chemistry introduces tools and methods—titration, chromatography, spectroscopy—that identify substances at trace levels. A gas chromatograph can break down complex mixtures into distinct peaks, letting inspectors verify water purity or confirm a product’s authenticity. Spectroscopic techniques, such as infrared or ultraviolet-visible analysis, reveal functional groups or concentrations by interpreting how samples interact with electromagnetic radiation.
This careful observation resonates across numerous sectors. Food manufacturers verify that raw materials comply with standards, ensuring no hidden contaminants. Forensic investigators rely on methods like mass spectrometry to link chemical residues to specific incidents or individuals. Doctors run blood tests, measuring hormone levels or searching for disease markers that guide diagnoses. Analytical chemistry’s principles emphasize precision, reproducibility, and thorough data interpretation, providing confidence in the conclusions drawn from each test.
Materials Science and Innovation
Materials science merges physics, chemistry, and engineering to design substances that exhibit desired mechanical, electrical, or thermal traits. Metals, ceramics, polymers, and composites each derive distinct properties from atomic-scale interactions. Alloying steel with elements such as chromium can enhance corrosion resistance, making stainless steel suitable for medical tools or kitchen utensils. Transparent ceramics hold promise for bulletproof windows and advanced laser systems because of their robustness and light transmission.
Nanotechnology, which manipulates substances at the atomic or molecular scale, seeks to harness quantum effects and molecular precision. Nano-coatings, for example, can repel water or resist bacteria on surfaces as different as smartphone screens and hospital furnishings. Light-emitting diodes and quantum dots underscore the synergy between electronic structure and macroscopic applications. By carefully engineering how atoms bond, materials scientists unlock advanced composites for aerospace, flexible electronics for wearable devices, or medical implants with specialized functions.
Chemical Safety and Precautions
Each stride in chemistry brings the necessity of careful handling and disposal of chemicals. Material Safety Data Sheets, labeling standards, and protective equipment collectively reduce the likelihood of accidental harm. Knowing how to manage corrosive acids, flammable solvents, or toxic byproducts helps protect technicians, researchers, and the environment. Laboratory training involves thorough hazard assessments, ensuring that emergency protocols stand ready for chemical spills or unexpected reactions.
Even in household settings, everyday items such as cleaning solutions, adhesives, or gardening supplies carry instructions aimed at preventing mishaps. Dilution guidelines help maintain safe concentrations, while recommended ventilation ensures fumes do not accumulate. Disposal practices, whether for expired medications or leftover paint, rest on an understanding of which substances can be neutralized or require special collection. With a deliberate balance between chemistry’s capabilities and protective measures, it becomes possible to enjoy technological and scientific progress without harming individuals or habitats.
Wrapping It Up
These core chemistry areas—atomic structure, reaction mechanics, stoichiometric balances, acids and bases, and beyond—tie together to shape how materials are created, used, and regulated. Each specialized topic builds on the previous one, crafting a cohesive scientific tapestry that continues to evolve. Organic innovations influence new manufacturing techniques, while electrochemical breakthroughs enhance portable power. Environmental considerations spark greener methods that cut pollutant generation and conserve natural resources.
In daily routines, chemistry reveals itself in the flavors that emerge from a kitchen pot, the cleansers that brighten a countertop, and the digital devices resting on desks or in pockets. Research institutions explore how chemical signals orchestrate cell activities, how catalysts might cut down industrial pollution, or how advanced polymers can lighten vehicles. Industries trace these discoveries to produce goods that are safer and more efficient, while healthcare experts align chemical insights with interventions that elevate patient well-being.
Mastering chemistry does not end with memorizing facts; rather, it involves forming a critical perspective about what materials do, how they transform, and how those transformations can be steered for positive results. From controlling reaction conditions to designing self-healing plastics or cultivating eco-friendly fertilizers, chemistry stands as a driving force that both clarifies the molecular domain and shapes the innovations poised to change everyday life.