Modern Physics – Relativity, Quantum Theory, and the Universe
Modern physics covers the theoretical breakthroughs and experimental findings that emerged after classical frameworks showed limitations. Researchers in the early 20th century and beyond developed models to describe very small scales, very high speeds, and very large gravitational fields. These advances include relativity, quantum theory, nuclear physics, particle physics, and cosmology, each reshaping our ideas of space, time, energy, and matter. Although these topics stretch far, the sections below provide a thorough guide to the core concepts, influential experiments, and practical applications.
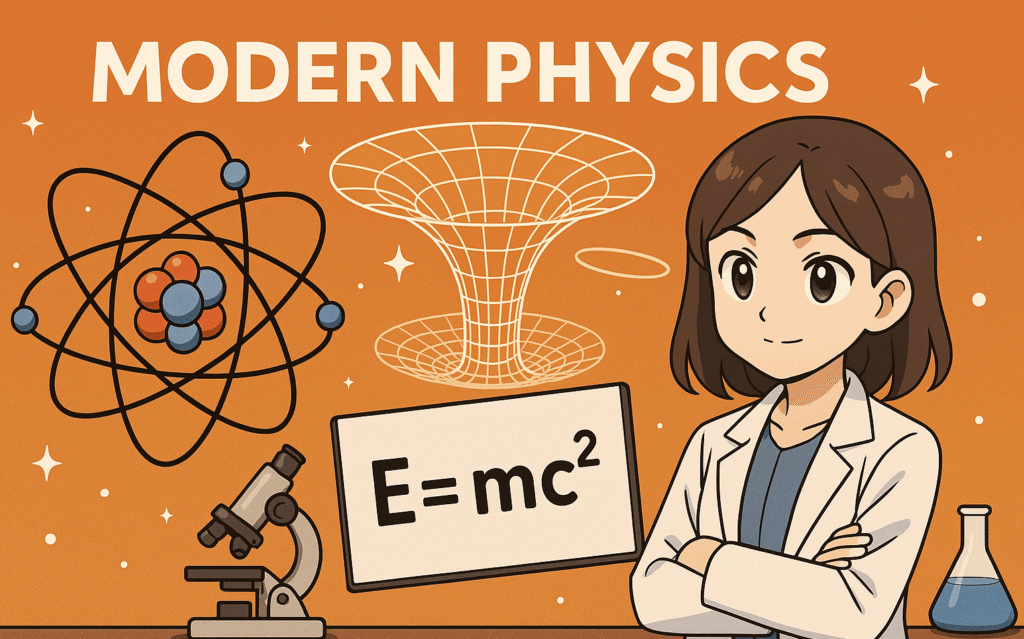
1. Introduction
Early physics relied heavily on Newton’s laws and Maxwell’s electromagnetism. Those models excelled at explaining a wide variety of everyday scenarios, from planetary orbits to electromagnetic waves. Yet, around the end of the 19th century, certain observations challenged these established views. The photoelectric effect, blackbody radiation, the stability of atoms, and peculiar results of measuring the speed of light all signaled that deeper principles were needed.
Physicists such as Max Planck, Albert Einstein, Niels Bohr, and Erwin Schrödinger contributed to the theoretical structure we now term “modern physics.” Einstein showed that space and time are not absolute but interwoven, leading to the special theory of relativity. He then extended those insights into the nature of gravity through general relativity. Meanwhile, Planck’s idea of energy quanta, combined with further work by Bohr, Heisenberg, Schrödinger, and many others, gave birth to quantum mechanics. These frameworks resolved perplexing data about atomic emission spectra, wave-particle duality, and chemical bonding.
By the mid-20th century, a more comprehensive quantum field theory approach emerged, describing electromagnetic and other fundamental interactions in a single formalism. The atomic nucleus also became a central area of inquiry, with nuclear fission, fusion, and radioactive decays revealing essential processes in stars and potential for controlled power generation on Earth. Particle accelerators probed deeper into matter’s structure, identifying a growing zoo of particles and gradually uncovering underlying constituents. Modern physics extends its reach outward as well, informing cosmological models that explain the origins and large-scale evolution of the universe.
The following sections introduce the major aspects of modern physics, highlighting conceptual breakthroughs, mathematical underpinnings, and real-world implications. This knowledge forms a bridge between the classical picture of the 19th century and the advanced perspectives shaping current science and technology.
2. Historical Background and the Shift from Classical to Modern
By the late 1800s, it seemed that classical physics offered comprehensive explanations of nature. Newtonian mechanics addressed force and motion for objects from falling apples to planetary bodies. Maxwell’s equations unified electricity and magnetism, clarifying electromagnetic wave propagation. Thermodynamics gave methods to handle heat transfer, efficiency, and energy conservation. Yet anomalies began to surface:
- Blackbody Radiation: Classical theories predicted that objects at thermal equilibrium would radiate infinite energy at short wavelengths, known as the “ultraviolet catastrophe.” This contradicted experiments.
- Photoelectric Effect: Observations showed that shining light on a metal surface could eject electrons, but classical wave ideas could not explain why only light above a certain frequency caused emission or why the energy of ejected electrons scaled with frequency rather than intensity.
- Atomic Stability: The classical electromagnetic framework implied that orbiting electrons in atoms should radiate away energy, spiral into the nucleus, and cause the atom to collapse. Real atoms do not behave this way.
- Michelson-Morley Experiment: Attempts to detect motion relative to a supposed “ether” failed, suggesting that the speed of light was constant for all inertial observers.
Physicists realized these conundrums demanded a fundamental revision of their theoretical constructs. Planck’s quantum of action bridged the gap in blackbody radiation analysis, while Einstein extended these quantum insights to light quanta (photons) in explaining the photoelectric effect. Models of atomic structure began to incorporate quantized electron orbits, addressing the problem of atomic stability and explaining emission spectra.
Further developments in relativity shifted the perception of space, time, and simultaneity, and eventually led to the concept that gravity is not merely a force but a geometric manifestation of spacetime curvature. Altogether, these transformations represent the core shift from the deterministic, continuous worldview of classical physics to the probabilistic, often counterintuitive realm that is modern physics. Although the classical frameworks remain accurate for many macroscopic, low-speed conditions, new models became essential to tackle phenomena at very small scales, very high velocities, or very intense gravitational fields.
3. Relativity
3.1. Special Relativity
Albert Einstein’s 1905 special relativity builds on the principle that the laws of physics hold in all inertial frames and that the speed of light in a vacuum is the same for all observers. These postulates lead to consequences that contradict common intuitions:
Time Dilation: Moving clocks tick more slowly when viewed from a stationary reference frame. If a spaceship travels near the speed of light, less time passes on board relative to Earth.
Length Contraction: A fast-moving object’s length along the direction of motion appears shorter to external observers.
Relativity of Simultaneity: Events that are simultaneous in one frame might occur at different times in another frame.
Mass-Energy Equivalence: The well-known equation indicates that mass and energy are interchangeable forms. A small mass can correspond to large energy if fully converted.
These outcomes resolve the null result of the Michelson-Morley experiment and unify the descriptions of mechanics and electromagnetism at high velocities. The Lorentz transformation replaces the Galilean transformation for velocity addition and time/space measurements, ensuring consistency with constant light speed.
3.2. General Relativity
A decade later, Einstein extended these ideas to include gravity. General relativity treats gravity not as a force but as a manifestation of curved spacetime caused by mass-energy distributions. The core equation, known as Einstein’s field equation, relates the energy-momentum content of spacetime to its curvature.
Key predictions include:
- Gravitational Time Dilation: Clocks run differently depending on gravitational potential.
- Orbital Precession: Mercury’s orbital path shifts in a manner that Newtonian gravity cannot fully explain.
- Bending of Light: Light passing near massive objects follows curved paths, verified experimentally during a 1919 solar eclipse.
- Gravitational Waves: Ripples in spacetime generated by accelerating masses. Detected directly for the first time in 2015 when two merging black holes emitted observable gravitational wave signals.
General relativity provides a framework for cosmic-scale phenomena, describing black holes, neutron stars, and the expansion of the universe. It combines elegantly with special relativity but remains challenging to unify with quantum theory at the smallest scales. Nonetheless, it has been confirmed by precise measurements in spacecraft orbits, GPS satellite operation (which requires relativistic corrections), and gravitational wave observatories. Together, special and general relativity replace many classical gravitational concepts with a geometry-based viewpoint that is indispensable for advanced astrophysical research.
4. Quantum Mechanics
Quantum mechanics emerged in the early 20th century to address discrete energy levels, wave-particle duality, and the probabilistic nature of subatomic behavior. Traditional classical physics had no explanation for phenomena like stable electron orbits, atomic emission spectra, or the photoelectric effect. Early quantum models, proposed by Planck and Bohr, introduced quantized energy states, while de Broglie suggested that matter particles can have wavelike properties.
4.1. Wavefunctions and Operators
The mathematical structure of quantum mechanics revolves around the wavefunction . The squared magnitude
represents the probability density of finding a particle at position
at time
. The Schrödinger equation,
governs how evolves, with
as the Hamiltonian operator encoding kinetic and potential energies. Observables like momentum or energy correspond to operators that act on
. Measurement yields eigenvalues, and outcomes generally follow a probabilistic interpretation.
4.2. Heisenberg Uncertainty Principle
Werner Heisenberg’s principle states that one cannot simultaneously measure certain pairs of conjugate variables (e.g., position and momentum, energy and time) with arbitrary precision. Instead, . This challenges the deterministic approach of classical physics, embedding probability and uncertainty at nature’s core.
4.3. Quantum Tunneling and Superposition
Quantum systems can exhibit tunneling, where a particle crosses a potential barrier it does not have enough energy to surmount classically. This underpins phenomena like alpha decay in radioactive nuclei and operation of tunneling diodes in electronics. Superposition lets quantum states add together, leading to interference effects. A particle can occupy a combination of states until a measurement forces a collapse to a definite outcome.
4.4. Applications
Quantum mechanics underlies atomic and molecular structure, explaining chemical bonding and periodic table organization. Solid-state physics draws heavily on band theory rooted in quantum ideas, enabling semiconductor design and microelectronics. Lasers rely on stimulated emission, predicted by quantum theory. Magnetic resonance imaging (MRI) exploits quantum spin alignment in strong magnetic fields. Without quantum mechanics, these devices and analyses would not function as they do. Although sometimes counterintuitive, quantum principles provide unmatched predictive power for microscopic phenomena.
5. Quantum Field Theory and Particle Physics
Classical field theories, such as Maxwell’s equations for electromagnetism, treat fields as continuous. Quantum field theory (QFT) extends this concept by describing particles as excitations of underlying fields. Each fundamental particle type corresponds to a field filling all of spacetime, and interactions manifest when these fields exchange quanta.
5.1. Birth of Quantum Field Theory
Attempts to reconcile special relativity with quantum mechanics led to early quantum electrodynamics (QED), which deals with electrons, positrons, and photons. QED calculations often involve Feynman diagrams, representing interactions as lines and vertices in spacetime. This formalism accurately predicts phenomena like the electron’s magnetic moment, matching experiments to extraordinary precision.
5.2. The Standard Model of Particle Physics
Over decades of experimentation, a framework known as the Standard Model emerged, encompassing:
- Quarks: Constituent elements of hadrons (e.g., protons, neutrons).
- Leptons: Electrons, muons, taus, and their corresponding neutrinos.
- Gauge Bosons: Force carriers (photons for electromagnetism, W and Z for the weak interaction, gluons for the strong interaction).
- Higgs Boson: Associated with the Higgs field, giving mass to certain particles through spontaneous symmetry breaking.
The strong, weak, and electromagnetic interactions are unified within the Standard Model’s gauge group structure, although gravity remains outside this picture. Particle accelerators like the Large Hadron Collider (LHC) probe energy scales high enough to produce short-lived heavy particles, checking for signs of physics beyond the Standard Model. The 2012 discovery of the Higgs boson confirmed a key prediction from the 1960s.
5.3. Ongoing Mysteries
Despite its success, the Standard Model leaves questions open. It cannot account for dark matter or the matter-antimatter asymmetry in the cosmos. Neutrino masses suggest physics beyond the Standard Model, since neutrinos were once assumed massless. Grand Unified Theories and supersymmetry are among the proposals aiming to integrate these puzzles, but no definitive experimental proof has emerged so far.
5.4. Practical Aspects
Particle physics research drives technology in unexpected ways. Accelerators developed for fundamental studies led to spinoffs like medical particle therapy and advanced imaging techniques. Detectors designed to track subatomic collisions helped develop improved sensor technology. High-energy experiments require massive computing resources, pushing data-processing innovations that later spread to broader scientific and industrial fields. Even when the mysteries of fundamental fields remain unsolved, exploration yields both deeper understanding and technical progress.
6. Nuclear Physics and Energy
Atoms contain dense nuclei made of protons and neutrons, which are themselves composite particles consisting of up and down quarks bound by the strong nuclear force. Nuclear physics delves into the behavior, stability, and transformations of these nuclei.
6.1. Radioactivity
Henri Becquerel’s accidental discovery of radioactivity (in 1896) revealed that some nuclei emit particles or radiation spontaneously. Three main types of decay are commonly named alpha, beta, and gamma. Alpha decay involves helium nucleus emission, beta decay transforms a neutron into a proton or vice versa (with corresponding emission of an electron or positron), and gamma decay releases high-energy photons. These decays show that nuclei can transform into other elements, defying the old assumption of atomic indivisibility.
6.2. Nuclear Fission and Fusion
- Fission: Heavy nuclei (like uranium-235 or plutonium-239) can split into smaller nuclei when struck by a neutron, releasing energy and more neutrons in a chain reaction. Controlled fission reactions in nuclear reactors produce electricity on a large scale, though handling radioactive waste and ensuring safety remain major concerns.
- Fusion: Lighter nuclei (like hydrogen isotopes deuterium and tritium) can fuse at high temperatures and pressures, forming helium and releasing energy. Fusion powers stars such as the Sun. Achieving sustainable fusion on Earth is challenging, requiring reactor designs like tokamaks or inertial confinement setups. If realized, fusion could offer a cleaner energy source with abundant fuel.
6.3. Applications
Beyond power generation, nuclear physics underpins techniques like radiocarbon dating, used in archaeology to determine artifact age. Medical imaging and treatments rely on radioactive isotopes, from PET scans to targeted radiotherapy for cancer. Industry employs radioactive tracers to gauge material wear or detect leaks. Overall, nuclear knowledge has influenced energy policy, medical diagnostics, and scientific research around the globe.
7. Astrophysics and Cosmology
Modern physics extends to cosmic scales through astrophysics and cosmology, exploring the life cycles of stars, the structure of galaxies, and the history of the universe itself.
7.1. Star Formation and Evolution
Nebulae rich in gas and dust can collapse under gravity to form protostars. If conditions permit nuclear fusion in the core, a stable star ignites. The Sun, for instance, fuses hydrogen into helium, releasing energy that radiates outward. Over time, stars evolve: high-mass ones burn fuel rapidly and may explode as supernovae, scattering heavier elements into space. Lower-mass stars endure longer, eventually becoming white dwarfs or neutron stars.
7.2. Neutron Stars and Black Holes
Neutron stars pack enormous mass into a small radius, composed mostly of neutrons. Their density is extreme, and their strong magnetic fields produce pulsar emissions. Black holes arise when stellar remnants with sufficient mass collapse so severely that not even light can escape. General relativity predicts an event horizon surrounding this region. Observations of gravitational waves from black hole mergers, along with imaging of black hole shadows (as in the Event Horizon Telescope’s landmark data), bolster the theory’s predictions.
7.3. Big Bang Cosmology
The large-scale structure of the universe suggests it began in a hot, dense state and has been expanding ever since. The Friedmann-Lemaître-Robertson-Walker metric, derived from general relativity, forms the backbone of modern cosmological models. Key evidence includes:
- Cosmic Microwave Background (CMB): Faint radiation pervading space, a remnant of the early hot universe.
- Abundance of Light Elements: Predictions of primordial nucleosynthesis match observed helium and deuterium levels.
- Galactic Redshifts: Most galaxies recede from us, with velocity roughly proportional to distance (Hubble’s law).
7.4. Dark Matter and Dark Energy
Galactic rotation curves and gravitational lensing indicate the presence of non-luminous matter that outnumbers ordinary (baryonic) matter. The exact nature of this dark matter remains unknown, though candidates include weakly interacting massive particles (WIMPs) or axions. Meanwhile, observations suggest the expansion of the universe accelerates, attributed to a form of dark energy. Researchers use space-based and ground-based observatories to refine data, hoping to clarify these puzzling components that dominate the mass-energy content of the cosmos.
Modern astrophysics and cosmology blend relativity, quantum processes, nuclear physics, and observational techniques to chart a detailed cosmic timeline and structure. The same laws that govern atomic processes can shape galaxy clusters billions of light-years away.
8. Technological and Practical Spin-offs
Modern physics is not confined to abstract theories; it has prompted widespread innovations that drive technology forward and benefit daily life.
8.1. Semiconductor Electronics
Quantum mechanics explained why materials have conduction and valence bands, allowing engineers to control electron flow with doping. Transistors, integrated circuits, and diodes emerged, ushering in the era of modern computing. Devices such as field-effect transistors rely on quantum tunneling principles, while semiconductor lasers appear in countless communication and sensing applications.
8.2. Lasers and Photonics
Stimulated emission, predicted by Einstein in 1917, paved the way for lasers. These devices produce coherent, monochromatic light used in optical storage (CDs, DVDs), fiber-based data transmission, bar-code scanners, and medical treatments (such as laser eye correction). Developments in nonlinear optics enable frequency doubling or parametric generation of new wavelengths, expanding the range of laser applications.
8.3) Nuclear Technology
Fission reactors provide electricity in numerous countries, albeit with concerns over safety, waste management, and proliferation risks. Radioisotopes serve as tracers in agriculture or as diagnostic agents in medicine. Radiography uses high-energy beams from radioactive sources to inspect structural integrity in engineering. Fusion research, though still in an experimental stage, holds promise for a cleaner long-term energy option if technical challenges can be overcome.
8.4. Medical Imaging and Diagnostics
Quantum physics insights led to the development of modern imaging techniques. PET scans rely on positron-emitting isotopes, while MRI exploits nuclear spin alignments in magnetic fields. X-rays, initially a 19th-century discovery, are now refined through advanced detectors and digital processing. These tools allow clinicians to visualize and diagnose internal conditions with far greater detail than older methods.
8.5. GPS and Satellite Systems
Relativistic effects matter for precise satellite-based navigation. GPS satellites travel quickly and orbit in weaker gravitational fields than Earth’s surface, meaning their onboard clocks run differently compared to clocks on Earth. Engineers use general and special relativity corrections to ensure accuracy down to a few meters or even centimeters with advanced techniques. This technology supports everything from smartphone mapping to synchronized power-grid operations.
Modern physics research consistently expands the range of possibilities for electronics, materials, energy production, and medical therapies. The link between theoretical breakthroughs and tangible devices underscores the strong interplay between scientific inquiry and practical development.
9. Philosophical and Interpretational Aspects
Modern physics challenges long-held ideas of deterministic predictability and absolute references for space and time. Quantum mechanics, with its probabilistic interpretation, raises questions about whether an underlying reality exists independently of measurements, or if the wavefunction collapse reveals a fundamentally observer-dependent process. Debates between the Copenhagen interpretation, many-worlds approaches, Bohmian mechanics, and other variants show that philosophical issues persist in quantum theory, even though all produce the same predictions in standard scenarios.
Relativity upends the notion of absolute simultaneity, implying that events depend on observers’ frames of reference. This challenges everyday thinking about time as universal or unchanging. The geometry-based view of gravitation in general relativity suggests that space and time themselves can be dynamic entities subject to curvature. These shifts resonate with philosophical inquiries into the nature of reality and knowledge.
Some efforts aim to unify general relativity with quantum mechanics under a single framework such as string theory or loop quantum gravity. These approaches might redefine spacetime at the Planck scale, bridging cosmic geometry with quantum fields. Whether this unification is simply a technical puzzle or a deeper conceptual revolution remains to be seen. Regardless, the legacy of modern physics indicates that scientific advances often transform fundamental assumptions, sparking ongoing dialogue between experiment, theory, and broader philosophical reflection.
10. Summary and Future Directions
Modern physics redefines how we view matter, energy, space, and time, replacing older absolute notions with flexible frameworks that accommodate high-speed or subatomic phenomena. Special and general relativity handle motion at relativistic speeds and gravitational effects on spacetime. Quantum mechanics, with its inherently probabilistic treatment, clarifies atomic and subatomic processes, explaining wave-particle duality, discrete energy levels, and entanglement. Quantum field theories offer a unified language for particles as field excitations, culminating in the Standard Model of particle physics. Nuclear research probes the tightly bound structures within atoms, unveiling radioactive decay, fission, and fusion. Astrophysics and cosmology project these theories onto cosmic scales, describing star formation, black holes, dark matter, and the universe’s evolution from a hot, dense beginning.
Technology benefits immensely from these theories. Semiconductor devices, lasers, nuclear medicine, and more illustrate the practical payoff of fundamental insights. Relativity-based corrections ensure precision in satellite navigation. Particle accelerators designed for research yield spin-offs in diagnostics and imaging. Ongoing questions, such as the nature of dark matter or the path to quantum gravity, show that modern physics remains far from complete. Multinational experiments and advanced observatories continue to refine measurements, driving a cycle of theoretical updates and experimental testing.
Looking ahead, bridging quantum mechanics with general relativity stands as a major theoretical goal. Observational efforts focus on gravitational waves, cosmic background radiation, and high-energy collisions to uncover hidden aspects of reality. The lessons from the 20th century’s revolutions in physics suggest that the universe’s deepest workings often defy traditional notions, and further transformation may emerge as new data arrives.